Abstract
After decades of basic, translational, and clinical research, cartilage regeneration therapies are still anticipated to impact the clinical landscape consistently. However, biological joint resurfacing and the regeneration of functional, integrated cartilage capable of wholly and permanently restoring joint function is still unavailable. Albeit structurally relatively simple, the cartilage extracellular matrix has a complex function in joint organ biomechanics, developmental processes, and adult life tissue turnover, repair, disease, and aging. The intricated cross-talk extracellular matrix-cellular component and its bidirectional role in maintaining tissue homeostasis are only beginning to be elucidated. Magnetic responsive nanomaterials (MNMs) have proven to be versatile tools for drug delivery, scaffold fabrication, alignment, and orienting cell distribution and cell fate. Due to their responsiveness to an applied magnetic field, MNMs can be remotely actuated for scaffold cell positioning, scaffold fabrication, or the delivery of touchless mechanical stimulation. In addition, MNMs possess excellent biocompatibility and are degradable through cellular iron storage metabolic pathways. Current directions for using MNMs to augment tissue engineering that recommends them as essential tools for fabricating bio-functional cartilage grafts are presented and discussed.
Introduction
Traumatic or degenerative articular joint diseases still represent an unsolved medical problem. Numerous scientists and clinicians invest consistent effort to deepen their understanding of the pathogenic mechanisms involved in joint decay, aiming to establish disease-modifying therapies. However, up to date, available therapeutic options oscillate between providing temporary symptomatic relief and radical suppression of the source of suffering by prosthetic joint replacement. None of these options offers an actual cure or provides true joint recovery. In the last decades, the regenerative medicine (RM)1 concept is sought to enable the anatomical and functional restoration of tissues and organs by manipulating developmental cellular and molecular events. RM has launched the quest for finding the most appropriate cell sources, supporting materials, and bioactive molecules that could completely restore a damaged joint surface2.
Biological joint resurfacing has become the subject of intense basic, preclinical, and clinical research3. Using in-depth knowledge of developmental events, RM is set to recreating tissues and systems endowed with all required properties. Strategies range from manipulating cells, genes, or de novo engineering biomimetic structures that can successfully replace, augment or even substitute abnormal, malfunctional, or missing ones. This distinction is necessary, especially considering general musculoskeletal tissues and joint components. Joints have a crucial role in providing and maintaining the movement of the skeletal pieces and are responsible for multilevel body interaction with the surroundings. They are composed of a large extracellular matrix (ECM) that can be as high as 98% of dry weight in the case of articular cartilage (AC)4.
In the past, ECM has been considered mainly as an inert structure that enables musculoskeletal pieces to accomplish their supportive role. However, ECM is increasingly recognized to play a crucial role in developmental processes and in adult life tissue turnover, repair, disease, and aging5. Furthermore, ECM has been identified as one of the crucial microenvironmental factors determining cell fate, influencing normal versus malignant6 or quiescent versus active stem cell phenotype7. Therefore, any attempt to recreate truly functional tissues, especially joint parts, should possess a clear perspective on the qualitative and quantitative parameters of engineered ECM.
An interesting development regarding bioengineering functional matrices is represented by incorporating magnetic nanomaterials (MNMs). MNMs can be employed as a drug and/or bioactive molecule delivery system as a means to control ECM alignment during fabrication as well as for the delivery of remote actuation for mechanical stimulation of differentiating cells. The main advantage of MNMs is that they are magnetic responsive and, therefore, controllable with magnetic field (MF) exposure. Magnetic responsiveness allows for the touchless manipulation of MNMs and the inert or biological structures that incorporate them. Furthermore, as MNMs are composed chiefly of iron-based materials, MNM is biodegradable by existent cellular and molecular pathways involved in iron metabolism.
In the following sections, ECM composition and functional roles will be outlined. Then, several aspects of the ECM’s role in joint development, disease, and aging will be further reviewed, followed by an overview of regenerative strategies that employ MNM as components for cartilage-engineered structures.
Structure and function of the articular cartilage ECM
Joints are the mobile pieces of the skeleton that connect bony segments conferring the mobility and stability required for performing various functions. Joints can be classified by considering their structure (modality of bone connection), function (degrees of mobility), biomechanical properties, or anatomical location within the body. From the structural perspective, the large majority of joints within the mammalian body are synovial (diarthrodial) joints8. Synovial joints account for an extensive range of motion and are distributed all over the body but mainly within the mobile segments, i.e., the limbs. Generally, a synovial joint is composed of two bony endings, each consisting of an enlarged part of cancellous bone, the epiphysis, covered with a thin layer of cortex named the subchondral plate9.
Long regarded as the most significant compound of the synovial joints and the main target of degenerative processes, AC forms the joint surface, the site of actual joint motility. AC is an ultra-specialized tissue composed mainly of ECM, with a high-water content (68 — 80%) and low amount (up to 2%) of specialized cells, the chondrocytes. The cartilage matrix comprises several distinct regions based on cell and ECM fibber arrangement: superficial, radial, deep, and calcified or subchondral zones (Figure 1). Depending on ECM’s relationship to the resident chondrocytes, the pericellular matrix is immediate to the chondrocytes, while the interterritorial matrix compartment represents ECM more distanced relative to the cellular component10. Collagens and PG account for the remaining dry weight with a small amount of lipids, phospholipids, non-collagenous proteins, and glycoproteins11. Collagen is the most abundant fibrous protein in AC, with 90 — 88% being type II and a small proportion of types I, IV, V, VI, IX, and XI. The extensive collagen fibers crosslinking and the different zone-dependent architecture account for the impressive biomechanical properties of the tissue.
The four zones of AC visible by light microscopy, namely superficial or tangential, intermediate or transitional, deep or radial, and calcified, are characterized by their specific collagen fiber orientation and specific placement of chondrocytes12. Transmission electron microscopy (TEM) shows that collagen fibers form a random network comparable to other tissues. However, a preferred zone-specific fibril pattern can be identified at a macroscopical and ultrastructural level. In the superficial zone (SZ), thin collagen fibers run parallel to the joint surface, and bigger fibril diameters and a radial disposition are characteristic of deeper zones. On the other hand, calcified cartilage (IZ) organize almost perpendicular to the plan of the subchondral bone. Furthermore, collagen IX covalently linked to the surface of type II fibers is thought to increase the network’s mechanical resistivity and provide restraint for proteoglycan molecules responsive to osmotic pressure. Collagen XI can be found primarily within the pericellular network displaying high affinity binding sites for PG (such as heparan and heparan sulfate)13.
Collagen type III co-localize with type II bundles in a small but constant ratio in normal human cartilage. In the osteoarthritic cartilage, collagen III produced by the chondrocytes is concentrated in SZ and upper zones, possibly similar to type I production as a response to matrix injury in other tissues14. Collagen type VI is localized predominantly in the chondrocyte pericellular space (Chodron) of adult AC. It has been found to increase during osteoarthritic progression and plays a functional role in cellular microenvironment remodeling associated with cartilage degradation15.
After cessation of skeletal growth, collagen type II synthesis by articular chondrocytes is dramatically reduced but not entirely absent. In mature animal models (dogs), its synthesis can be increased up to 10-fold in response to injury16. Based on synthesis rates of hydroxyproline, overall collagen compound turnover in adult humans has been calculated to be around 400 years for AC of femoral heads17. It is possible that subfractions of collagen compounds, such as SZ or pericellular matrix, display different turnover rates. The fact that collagen fiber diameter increases with age support that AC has a turnover and is remodeled during an individual lifetime. Chondrocyte appears to be involved in controlling the synthesis and assembly of various collagen polymer fibers. In turn, chondrocyte survival during adulthood and aging processes depends on ECM structure and biomechanics18, resulting in a deterministic interrelation between the cell compartment and AC ECM that is dynamic and interdependent.
Regarding proteoglycans, a significant part of those present in AC is the large chondroitin sulfate molecule known as aggrecan. Aggrecan displays a unique feature; when the core protein interacts with another glycosaminoglycan (GAG), hyaluronan (HA) forms a particularly strong interaction with high binding affinity. Another characteristic of this bond is the presence of a link protein (LP) homologous to the aggrecan core protein’s globular region that binds both the aggrecan core protein and HA. The linkage of aggrecan, link protein, and HA is non-dissociating and non-displaceable under physiological conditions. It is regarded as one of the hallmarks of chondrogenesis and one of the leading causes of its remarkable physical stability. Aggrecan molecules are present within cartilage as proteoglycan aggregates, each composed of a central filament of HA with up to 100 aggrecan molecules radiating from it, stabilized by the LP.
Proteoglycan aggregate structure is influenced by three parameters: the length of the HA, the proportion of link protein, and the degree of aggrecan processing. In addition, other proteoglycans have been identified in cartilage, such as small leucine-rich repeat proteoglycans common to other tissues (decorin, biglycan, perlecan, fibromodulin, and lumican). During cartilage development, proteoglycans seem to exert essential functions during condensation and chondrocyte differentiation and growth stages.
Temporal and spatial patterning of the heparan sulfate proteoglycans influences the presentation of the heparin-binding growth factors to their signaling receptors involved in anlagen growth and differentiation19. Normal cartilage function depends on a high cartilage aggrecan content, high GAG substitution, and large aggregate size. Aggregates account for tissue turbidity and provide the osmotic properties necessary to resist compressive loads and retain water. Small leucine-rich repeat proteoglycans also maintain the integrity of the tissue and modulate its metabolism20. Loss of cartilage integrity in osteoarthritis is commonly associated with impaired aggrecan function due either to proteolytic cleavage of the aggrecan core protein, resulting in decreased aggrecan charge, or cleavage of the HA, which decreases the aggregate size.
Magnetic nanomaterials for ECM engineering
Cartilage ECM is the primary ultra-specialized structure that accounts for the remarkable endurance of the tissue to mechanical stress. However, beyond its mechanical function, ECM is a major player among the microenvironmental compounds that influences cell behavior. Significant cellular phenotype changes from proliferation, adhesion, and migration to differentiation and death are strictly correlated and guided by ECM structure, composition, and physical and chemical properties. A highly versatile structure, ECM is constantly remodeled during developmental processes exerting swift control over cell fate during this period of rapid and transient changes7. For example, during postnatal tissue growth and in adult tissue maintenance, cartilage ECM — chondrocyte cross-talk account for progressive tissue maturation and stabilization shown to be correlated with joint function21. Aging and significant joint pathological changes, such as osteoarthritis and inflammatory arthritis, affect the integrity of homeostatic ECM; aggrecan content is decreased, and collagen content is increased with a shift from collagen II to collagen I. Furthermore, ECM alteration predisposes the tissue to mechanical failure resulting in altered mechanical environments of the cells within the cartilage matrix22. Even though the knowledge regarding cell mechano-sensing was previously available, it is only recently that the importance of cell mechano-sensitivity is being explored at a molecular level. Dynamic studies regarding ECM-mediated availability of mechanical stimuli increased awareness about the bidirectional cross-talk ECM and ECM-producing cellular components.
Given the complex structural and functional role in cartilage development, growth, and maintenance, bioengineering a structural enduring and cell-instructing ECM is crucial for designing functional implantable tissues. Even though AC appears relatively simple, composed only of one cell type and ECM fibbers, obtaining functional implantable cartilage grafts has proved challenging23. Several issues related to graft integration within the host tissue, mechanical endurance and force distribution during mechanical stimulation, cell -engineered matrix cross-talk still need to be resolved. MNMs are regarded as multifunctional tools able to consistently enhance the functional engineering of cartilage24. Their versatile employment as carriers, enhancers of scaffold fabrication, and/or functionalization, separately or in combinations, offer appealing modalities for advancing functional cartilage engineering25.
Magnetic nanomaterials (MNMs)
Magnetic nanomaterials are nanosized materials of various structures, shapes, and compositions capable of responding to an applied MF. A MF gradient can be used to remotely position, filter, or arrange MNMs, MNM-containing composites, or even living cells. MNMs are composed mainly of metallic structure that preserves bulk material properties and develops a number of unique properties due to the small size, surface extension, or aspect ratio26. New and interesting properties can be obtained using bimetallic materials. The performance of these materials often exceeds those of simple metals by changing the metallic components, the morphology and structure of the nanomaterial change, and their physical and chemical properties. The magnetic behavior of MNMs depends on the type of material, surface characteristics, and MNM size. Due to the high surface-to-volume ratio, such structures offer an increased area for functionalization compared to their bulk counterparts. In order to counteract one of the major disadvantages of using MNMs, their poor stability and tendency to agglomerate, very small nanoparticles with superparamagnetic behavior are used9. A particular advantage of magnetic materials, especially for iron oxide-based structures, is that magnetite and maghemite have excellent biocompatibility. In order to avoid quick degradation and to increase the ability to interact with biological surroundings, the surface of the MNMs is usually coated with biocompatible organic compounds (e.g., polyethylene glycol (PEG) or dextran)27. The encapsulation of the magnetic nanoparticles (MNPs) in an organic compound layer increases the duration of circulation of the MNPs inside the body, making this type of nanomaterial highly biocompatible28.
MNMs are commonly categorized based on their shape, magnetic behavior, and/or composition. MNPs are spherical MNMs almost equal in their three-dimensional size (ranging from 5 - 100 nm) with a more bead-like appearance. MNPs can be prepared either by chemical co-precipitation or electrochemical synthesis, which is known to influence physic-chemical properties (Figure 2). Nanostructured Fe3O4 MNPs are most commonly known as magnetite or the oxidized form of maghemite29. MNPs based on Co, Ni, Fe, CoFe, NiCo, and NiFe can also be prepared; however, they display low biocompatibility and are, therefore, used less for applications that come in direct contact with the living matter. Preferably, MNPs sought for biomedical applications exhibit superparamagnetic properties (SPIONs)30. SPIONs are small-sized MNPs composed of magnetite (Fe3O4) or maghemite (γ-Fe2O3) core, usually coated or precipitated throughout a porous biocompatible polymer. Maghemite and magnetite are ferromagnetic. However, as the size of the particle decrease to 30 nm or lower, they become superparamagnetic31, thereby decreasing the risk of agglomeration and facilitating their use for biomedical applications.
Magnetic nanowires typically have two equal and one larger dimension (4 – 10 respectively 100 – 150 nm), their physical appearance resembling a nanoscale rod32. Nanowires containing noble metals and one of the transition metals are the most studied due to possible applications in biosensors miniaturization, drug delivery, separation manipulation, and as a substrate for culturing living cells (Figure 3)33, 34. Plate-like MNMs typically have two dimensions with increased size (20 – 600 nm) compared to the third (2 – 3 nm), giving them an appearance of a nano-scaled plate. Synthesis of plate-like nanoparticles is based on lithographic techniques, such as electron beam lithography, combined with electrodeposition or sputtering35. As a result, well-ordered nanoplates with diameters ranging between 20 and 600 nm and different thicknesses can be prepared. Furthermore, electrodeposition or sputtering can fill the nano-holes with different metals (Co, Ni, Fe, Au, Pt, and Ag) or alloys (NiFe, CoFe, CoPt). Like MNPs, octahedral or cuboid MNMs have all three dimensions almost equal. However, they physically resemble the geometrical shape obtained by chemical synthesis by modulating reaction conditions such as the nature and concentration of chemical reactants, temperature, or reaction pH36.
The remarkable advantage of using iron-based MNMs resides in their biocompatibility. Fe is a naturally occurring metal in all living organisms and is stored as ferritin in humans and mammalians. Cells use innate iron-handling mechanisms to metabolize iron-containing MNMs. Cells incorporating or attached to a MNM substrate demonstrate good viability, retaining their proliferative potential and specific phenotype (Figure 4). Issues regarding oxidative stress potentially induced by MNM degradation and the risk of iron overload must be carefully considered when testing MNMs in vivo37.
MNM in drug and/or bioactive molecule delivery
The concept of manufacturing scaffolds with built-in cues for cell engraftment, survival, proliferation, and differentiation emerged as a necessity to mimic the natural ECM active role in tissue maintenance aiming at functional engineering38. Several methods for scaffold-mediated controlled bioactive molecule or drug delivery have been tested with variable success for cartilage engineering. Hydrogels are primarily used as drug delivery carriers; however, their efficiency depends upon mesh size, swelling properties, and network degradation. Magneto-responsive hydrogels that can be implanted and manipulated to release drugs on-demand via MF exposure could potentially impact the field of cartilage engineering. Different hydrogel and magneto-responsive combinations have already been tested for their potential use in supporting chondrocyte or mesenchymal stem cell adhesion and viability. In a first attempt to obtain a magnetically responsive composite, a hybrid hydrogel containing type II collagen, HA, and PEG with incorporated magnetic nanoparticles was used to produce a magnetic nanocomposite hydrogel (MagGel) for cartilage tissue engineering. MagGel was shown to be successfully crosslinked and to respond to an external magnet while maintaining structural integrity in physiological fluids under remote magnetic guidance while allowing the adhesion of bone marrow-derived mesenchymal stem cells (BMMSCs)39.
In another approach, non-cell adhesive polyvinyl alcohol hydrogels were mixed with magnetite (Fe2O3) nanoparticles, HA, and chondroitin sulfate. HA and chondroitin sulfate blended hydrogel as natural polymers were shown to increase pore size and the equilibrium swelling ratio (ESR) while maintaining excellent compressive strength and promoting chondrocyte adhesion and growth40. Based on these findings, a hydrogel and MNM combination was used to deliver small molecules that support BMMSC chondrogenesis41.
Ultrasmall superparamagnetic iron-oxide (USPIO) MNPs functionalized with kartogenin have previously been shown to support chondrocytes’ BMMSC differentiation and integrated with cellulose nanocrystal/dextran hydrogels. Kartogenin was shown to undergo long-term stable, sustained release and could recruit endogenous host cells, inducing BMMSCs to differentiate into chondrocytes in both in vitro and in vivo models of cartilage defect in rabbits. Furthermore, due to the USPIO component, hydrogels exhibited a distinct magnetic resonance contrast detected by magnetic resonance imaging (MRI) in vivo and in vitro42. In addition, a model of MF-dependent drug delivery was shown to be efficient in repairing cartilage defects in a rabbit model. Compared to controls at 12 weeks after intraarticular injection, magnetic liposomal (magnetite, egg phosphatidylcholine, and cholesterol) containing TGF-beta1 facilitated by an external magnet was shown to accumulate within a cartilage defect, thereby enhancing cartilage repair43.
MNM for scaffold fabrication
MNMs were used in the process of fabricating supportive structures for cartilage engineering. MNMs crosslinked with synthetic or natural polymers in solution or in hydrogel form within a magnetic field were used as a modality to remotely control the formation of a desired structure44. By modulating magnetic material concentration, type duration, and polarity of MF exposure, scaffolds that closely mimic the native cartilage matrix could be obtained. As a proof of concept, in the absence of an external magnetic field, MNP crosslinked with different ECM molecules, natural or synthetic, are randomly dispersed in a liquid, as they have no net magnetic moments at room temperature. Upon introduction within a MF, the energy of interaction between the MF and MNP creates a magnetic moment that overcomes the thermal fluctuation energy, resulting in individual MNM magnetic moments orienting parallel to the direction of the MF. This results in their arrangement into chain-like aggregates, determining the formation of nanostructures approximating natural fiber distribution within ECM45.
Mixing alginate-coated iron oxide MNPs and alginate solution and exposure to MF during freezing could infer three classes of anisotropy within the resulting structure. Scaffolds with anisotropic surface topography, microstructure (as reflected by pore alignment), and mechanical properties (matrix stiffness) could be obtained using this method. As a plus, the scaffold retains magnetic responsiveness during cell seeding and culture, which can be used to deliver mechanical actuation during chondrogenesis46. Such a magnetically controlled assembly could be used to design structures with aligned nanofibers with several degrees of anisotropy in scaffold structure to further orient cell seeding and their subsequent growth and maturation.
A similar approach was used in obtaining supramolecular hydrogel structures tested in vitro for their efficiency in repairing cartilage defects. Bone marrow mesenchymal stem cell-laden supramolecular hydrogels administered via injection were shown to promote the regeneration of both hyaline cartilage and subchondral bone in the rat osteochondral defect model. This supports cell viability and improves hydrophilic and hydrophobic bioactive molecule delivery and targeting of diseased tissues47.
Magnetic handling was shown to help create layered, biomimetic scaffolds for cartilage TE. A three-layered nanocomposite hydrogel (ferrogel), with a gradient in compressive modulus from the top to the bottom layers, proved to possess a depth-dependent strain gradient. Such a construct could support bovine chondrocyte viability, and sulfated GAG expression increased with depth from the surface. By modulating the density of magnetic nanoparticles, concentration of hydrogel as well as cell number, such a biomimetic scaffold could be tailored to meet the physiological condition of the host48.
Several reports regarding the use of MNP and magnetic force have not been tested for cartilage regeneration but could offer interesting avenues in the future. For example, MNP and magnetic force could be used to create cell layers protective against immune response49 or as a modality to tune residues of arginine-glycine-aspartic acid motifs (RDG) dependent substrate anchoring of seeded cells50.
Magnetic scaffolds were shown to possess superior structural and mechanical performance for cell adhesion, cell proliferation, and osteogenic differentiation in vitro while promoting a higher amount of bone regeneration in an animal skull defect model51. These methods could also be tested for cartilage or osteochondral tissue repair in the future.
MNM in handling cells for cartilage TE
MNM has been used as a modality to improve the process of cell seeding and distribution within a scaffold or to form guided scaffoldless cell assemblies, such as spheroids or cell sheets. Magnetic force-based cell seeding could obtain cell condensation within a scaffold structure and improve mesenchymal stem cell-based chondrogenesis. Since a desired distribution of cells within the scaffold is often difficult to achieve due to the hydrophobic nature of most scaffold materials, using remote-controlled magnetic deposition of cells incorporating MNM, such as magnetic nanoparticles and magnetic cationic liposomes, could be an attractive modality to solve this issue. Seeding cells on 3D scaffolds makes it relatively easy to seed cells onto the surface layers. However, there is generally a relatively poor penetrability and cell density in the deeper layers of the scaffold. Non-magnetic approaches to solve these problems have been proposed, such as dynamic seeding methods and seeding within bioreactors. However, these methods have limitations in that they may either destroy the scaffold architecture or result in relatively thin structures. Magnetic tissue engineering (Mag-TE) has been proposed to circumvent these problems using magnetic responsive cationic liposomes attached to the surface of living cells. This approach was proven successful in populating commercially available PLGA scaffolds, with cell-seeding efficiency being enhanced from 58.9% to 10.8% compared to static seeding52, 53. In addition, the Mag-TE method was reported to be efficient in patterning cells in scaffoldless structures, layering hepatocytes and endothelial cells54, or obtaining structures from mesenchymal stem cell sheets that were successful in repairing calvaria bone defects in a rat model55. While no report involving cartilage TE that uses a similar method exists, the approach could inspire the field of chondral or osteochondral regeneration.
The idea of obtaining high cellular condensates that would mimic embryonic stages of cartilage development using magnetically manipulated cells has been tested by several groups with interesting results56. Magnetite-labeled MSCs were shown to condensate within a polysaccharide (pullulan/dextran) porous scaffold. When combined with further maturation within a dynamic bioreactor, cell condensates with up to 50% increase in collagen II and proteoglycan production were obtained57.
Handling cells using magnetic force was used for guided cell delivery within a cartilage defect. Magnetic targeted delivery of MSCs was reported to increase efficiency and decrease the number of cells required to obtain cartilage defect healing in animal models. Furthermore, compared to non-magnetic targeted delivery controls, autologous MNP-loaded MSCs guided with an external static MF were able to improve the repair of chronic large osteochondral defects in rabbits58 and patellar defects in minipigs59. Moreover, intraarticular placed magnets were used in addition to a modality to guide synovial-derived MSCs in osteochondral defects in rats60. Interestingly, magnetic targeted delivery and MF exposure proved to increase the expression of adhesion proteins in transplanted cells (integrin α2, integrin α6, and integrin β3)61, 62, possibly favoring intercellular communication and building of de novo formed ECM.
Further development of the method is needed since the application of external magnets is only restricted to several anatomical areas of larger joints, such as the knee, and is strongly influenced by the direction of the force and distance to the surface. Therefore, it would be limited to patellar or tibial plateau defects and problematic in bulkier patients. In addition, internally placed magnets would require a second surgery for removal, while their size can expose them to further cartilage damage.
Magnetically assisted formation of scaffoldless structures: cell sheets and spheroids
Using scaffoldless cellular aggregates as an alternate modality to approach cartilage TE has been shown to preserve cell phenotype and viability and provide an environment that mimics the embryonic stage of cell condensation during joint formation63. MNMs have been used to remote control cell organization into scaffoldless tridimensional structures. MSCs loaded with MNPs were magnetically actuated to form cellular spheroids. Cells were shown to self‐organize into constructs similar to corresponding tissues in vivo, with epithelial cells forming sheets while fibroblasts form spheroids. SEM demonstrated such constructs to reproduce intercellular connections64, 65. Magnetic levitation of cells in the presence of MNM has been shown to enable multicellular clustering and patterning of different cell types in co-culture, for example, hydrogel consisting of gold, magnetic iron oxide nanoparticles, and filamentous bacteriophage.
Furthermore, our group reported increased dynamic of spheroid formation, improved cell viability, and chondrogenic conversion in adipose-derived mesenchymal cells (ADSC) loaded with prepared magnetite, prospecting that this method could be scaled up to obtain TE cartilage66. Taking advantage of the magnetic responsivity induced by the uptake of various MNMs, cells can be layered in sheet-like structures. Such sheets can be further stacked and tailored to form custom-made 3D structures. The use of magnetic technology to obtain cell sheets (MagCs) has been reported to enable the formation of multicellular constructs that closely reproduce developmental microenvironments and generate complex structures that more closely reproduce native tissues. For example, MNP-loaded dental pulp epithelial cells and dental mesenchymal cells were layered as sheets using a magnetic force. Immunostaining revealed collagen IV formation and epithelial-mesenchymal interactions between cell sheets, possibly forming similar microenvironmental conditions than developing dental bud tissue67. Also, locally transplanted multilayered human MSCs containing magnetic liposomes were shown to achieve increased blood flow recovery and angiogenesis in a model of nude rat hind limb ischemia compared to injected MSCs attributable to increase expression of vascular endothelial growth factor combined with apoptosis68.
The magnetic responsivity of MagCs constructs can also be used to deliver continuous or intermittent stimulation during tissue maturation, thus increasing mechanical properties. Moreover, pulsed electromagnetic field stimulation delivered to human tendon cell sheets favored the expression of anti-inflammatory genes, increasing both the tenogenic and immunomodulatory properties of the construct69. Cell sheet technology was also used to layer chondrocyte and synovial cells, which proved successful in repairing AC defects70. MNMs can be functionalized to assist local regeneration by delivering bioactive molecules. Graphene oxide-coated MNPs (magnetite) functionalized with bone morphogenic protein 2 (BMP-2) and incorporated within dental pulp stem cells (DPSC) was shown to improve bone formation in vitro. The same team reported a combined osteochondral construct using DOSC loaded with MNP functionalized with BMP-2 for the osseous part and transforming growth factor beta 3 (TGFβ3) functionalized for the chondral part, respectively71.
MNM for directing cell fate
MNM has been used to increase the chondrogenic conversion of non-differentiated MSCs. Two aspects seem efficient in directing cell fate towards chondrogenesis: iron content and magneto-mechanical actuation facilitated by their presence inside or attached to the cell membrane. Commercially available iron oxide MNP labeling has been shown to increase chondrogenic phenotype and osteogenesis in chondrocytes seeded on a cultivated biphasic, type II collagen-chitosan/poly(lactic-co-glycolic acid) scaffold. Gene expression analysis showed increased aggrecan and collagen II expressions in chondrocytes and decreased SRY-related high mobility group box gene (Sox-9) associated with osteogenesis, respectively72. Further, magnetic nanoparticle biodegradation by human MSCs increased during in vitro chondrogenesis parallel with a decrease in magnetic responsivity, suggesting MNP biodegradation. MSC loaded with MNPs displayed downregulation of transferrin and upregulation of ferro-pontin genes during chondrogenesis but not during adipo and osteogenesis73. As a fact, ITS premix supplement containing insulin, transferrin, and selenium is primarily used for in vitro chondrogenesis and for long-term preservation of adult chondrocytes74. This paradoxical situation might be explained by the hormetic role of MNP present in switching metabolic pathways associated with chondrogenesis. Abolishing or limiting intracellular iron uptake might upregulate the transcription of chondrogenic Sox-975. The fact that iron supplementation is capable of upregulating chondrogenesis was demonstrated in vivo in animal models of cartilage repair. Bone marrow-derived MSCs pretreated with ascorbic acid and iron supplement ferumoxytol were shown to accelerate the repair of full-thickness cartilage defects in minipigs. This could be produced by reducing metal ions to a biologically active ferrous form, preventing free-radical mediated tissue damage, promoting proline and lysine hydroxylation, and supporting the formation of mature ECM collagen molecules76.
MNM-induced discrete mechanical stimulation could potentially be used to increase chondrogenesis by MSCs. Adipose-derived stem cells loaded with prepared MNP submitted to magneto-mechanical stimulation within alternating MFs were shown to increase chondrogenic conversion as assessed by increased GAG production. However, this effect appears to be cell type dependent since Wharton Jelly-derived MSCs could not increase GAG production in the same experimental conditions, mainly due to differences in MNP upload77.
MNM and additive manufacturing of cartilage
Layer-by-layer deposition of combined biological materials, cells, and biological factors is known as additive biomanufacturing. In recent years, many technical developments have employed additive manufacturing principles to engineer complex tissues and organs directly. Such an approach is sought to provide personalized solutions for cartilage repair by printing customized implantable grafts or even by allowing in situ printing at a certain point. 3D bioprinting hydrogels have significantly advanced the field of cartilage and bone tissue engineering. Using MNM could impact the field by introducing the ability to adjust the shape and structure of printed tissue using MF. Hybrid hydrogels containing MNPs were shown to support the growth and chondrogenic differentiation of BMSCs37. Therefore, similar hydrogels and cells could be used for bioprinting cartilage and/or osteochondral grafts78. In vitro tests have demonstrated that MNP incorporation within biopolymeric hydrogels (fibrin-agarose) and adult chondrocytes increased storage values and loss moduli with good cell viability, proliferation, and hydrogel swelling control79. Therefore, using such mixtures could impact the field of cartilage bioprinting. Using inks with different MNP compositions would enable 4D multi-material direct ink printing of soft magnetic actuators to be used to stimulate cells during graft maturation. Given that direct ink printing MNP-containing hydrogels exhibited macroscopic anisotropy, porosity, and good mechanical stability, they could offer an exciting solution for functional cartilage printing80.
Conclusion: advancing the field of cartilage ECM engineering using MNM
The concept of using magnetic responsiveness of cells and/or biomaterials is increasingly tested as a modality to upgrade the design and fabrication of biomimetic cartilage grafts. Many benchtops or in vitro studies demonstrate that MNM has exciting advantages, such as good biocompatibility, remote manipulation, the possibility for up-scaling manufacturing, and cost efficiency. Creative approaches to extending the use of magnetic behavior are emerging. A possible approach would be positioning diamagnetic objects in 3D hydrogel using MNPs. Rather than magnetizing the objects within the hydrogel, diamagnetic objects, such as polystyrene beads, drug delivery microcapsules, and living cells, were shown to be patternable in response to brief MF exposure. Upon photo-crosslinking the hydrogel precursor, object positioning is maintained while the MNP diffuses out of the hydrogel, supporting long-term construct shape81. Another development is the use of MNM-containing hydrogels for both the delivery of magneto-mechanical actuation and to sense the degree of mechanical stress applied to cells82. Of significant interest would be the creation of MNM-containing scaffolds that can sense multiple physical parameters, such as degradation rate, loading forces, and pH fluctuation. Such a sensing system would be able to inform clinical decision-making after implantation surgery.
Given the nanoscale dimension of the MNM used for modulating regenerative effects, further developments are expected to build upon the ability to modify cellular mechano-sensing pathways, enhancing the ability to produce and assemble structural proteins required for de novo matrix production. Correlating scaffold degradation with the rate of matrix build-up by implanted cells would improve graft stability and integration and potentially decrease recovery time post-implantation.
Finally, there is an increasing need for cross-talk between material scientists, physicists, cellular and molecular biologists, and clinicians from the initial stages of designing potential novel cartilage engineering approaches involving MNM. Sharing results through open-access databases and standardization of methods and equipment used for the fabrication and exploitation of MNM would be another obligatory step in furthering rapid translation to clinics of regenerative medicine products.
Abbreviations
Ag: silver, AC: articular cartilage, ADSC: adipose derived mesenchyma cells, BMMSC: bone marrow derived mesenchymal stem cells, BMP: bone morphogenetic protein, Co: cobalt, CT: computer tompgraphy, ECM: extracellular matrix, ESR: equilibrum swelling ratio, Fe: iron, GAG: glycosaminoblycan, HA: hyaluronic acid, LP: link protein, MF: magnetic field, MNM: magnetic(responsive) nanomaterials, Ni: Nickel, PEG: polyethylene glycol, SZ: superficial zone, SEM: scanning electron microscopy, SPION: Superparamagnetic iron oxide nanoparticles, TEM: transmision electron microscopy, TGF: transforming growth factor, USPIO: ultrasmall superparamagnetic iron oxide nanoparticles, 3D: three dimensions, 4D: four dimensions
Acknowledgments
Authors thank Dr Oana Dragos-Pinzaru for providing images of nanowire substrate and Dr P Plamadeala for providing histology images of normal cartilage.
Author’s contributions
DDH drafted and edited the MNM chapter and their use in cartilage repair, LL conceived and drafted the manuscript. All authors read and approved the final version of the article.
Funding
Financial support by Romanian Ministry of Research, Innovation and Digitization, CNCS/CCCDI-UEFISCDI, project number ERANET-EURONANOMED-3-OASIs, within PNCDI III (contract number 273/2022) is kindly acknowledged.
Availability of data and materials
Not applicable.
Ethics approval and consent to participate
Not applicable.
Consent for publication
Not applicable.
Competing interests
The authors declare that they have no competing interests.
References
-
Mason
C.,
Dunnill
P.,
A brief definition of regenerative medicine. Regenerative Medicine.
2008;
3
(1)
:
1-5
.
View Article PubMed Google Scholar -
Stem cell drugs: the next generation of pharmaceutical products. Biomedical Research and Therapy.
2016;
3
(10)
:
857-871
.
View Article Google Scholar -
Myers
K.R.,
Sgaglione
N.A.,
Grande
D.A.,
Trends in biological joint resurfacing. Bone & Joint Research.
2013;
2
(9)
:
193-9
.
View Article PubMed Google Scholar -
Sophia Fox
A.J.,
Bedi
A.,
Rodeo
S.A.,
The basic science of articular cartilage: structure, composition, and function. Sports Health.
2009;
1
(6)
:
461-8
.
View Article PubMed Google Scholar -
Lu
P.,
Takai
K.,
Weaver
V.M.,
Werb
Z.,
Extracellular Matrix Degradation and Remodeling in Development and Disease. Cold Spring Harbor perspectives in biology.
2011;
3
(12)
:
a005058
.
View Article PubMed Google Scholar -
Quail
D.F.,
Joyce
J.A.,
Microenvironmental regulation of tumor progression and metastasis. Nature Medicine.
2013;
19
(11)
:
1423-37
.
View Article PubMed Google Scholar -
Watt
F.M.,
Huck
W.T.,
Role of the extracellular matrix in regulating stem cell fate. Nature Reviews. Molecular Cell Biology.
2013;
14
(8)
:
467-73
.
View Article PubMed Google Scholar -
McNally
E.G.,
Ultrasound of the small joints of the hands and feet: current status. Skeletal Radiology.
2008;
37
(2)
:
99-113
.
View Article PubMed Google Scholar -
Yu
M.,
Huang
S.,
Yu
K.J.,
Clyne
A.M.,
Dextran and polymer polyethylene glycol (PEG) coating reduce both 5 and 30 nm iron oxide nanoparticle cytotoxicity in 2D and 3D cell culture. International Journal of Molecular Sciences.
2012;
13
(5)
:
5554-70
.
View Article PubMed Google Scholar -
Clockaerts
S.,
Bastiaansen-Jenniskens
Y.M.,
Runhaar
J.,
Van Osch
G.J.,
Van Offel
J.F.,
Verhaar
J.A.,
The infrapatellar fat pad should be considered as an active osteoarthritic joint tissue: a narrative review. Osteoarthritis and Cartilage.
2010;
18
(7)
:
876-82
.
View Article PubMed Google Scholar -
Sophia Fox
A.J.,
Bedi
A.,
Rodeo
S.A.,
The basic science of articular cartilage: structure, composition, and function. Sports Health.
2009;
1
(6)
:
461-8
.
View Article PubMed Google Scholar -
Eyre
D.,
Collagen of articular cartilage. Arthritis Research.
2002;
4
(1)
:
30-5
.
View Article PubMed Google Scholar -
Aigner
T.,
Bertling
W.,
Stöss
H.,
Weseloh
G.,
von der Mark
K.,
Independent expression of fibril-forming collagens I, II, and III in chondrocytes of human osteoarthritic cartilage. The Journal of Clinical Investigation.
1993;
91
(3)
:
829-37
.
View Article PubMed Google Scholar -
Chang
J.,
Poole
C.A.,
Sequestration of type VI collagen in the pericellular microenvironment of adult chrondrocytes cultured in agarose. Osteoarthritis and Cartilage.
1996;
4
(4)
:
275-85
.
View Article PubMed Google Scholar -
Eyre
D.R.,
McDevitt
C.A.,
Billingham
M.E.,
Muir
H.,
Biosynthesis of collagen and other matrix proteins by articular cartilage in experimental osteoarthrosis. The Biochemical Journal.
1980;
188
(3)
:
823-37
.
View Article PubMed Google Scholar -
Maroudas
A.,
Bayliss
M.T.,
Venn
M.F.,
Further studies on the composition of human femoral head cartilage. Annals of the Rheumatic Diseases.
1980;
39
(5)
:
514-23
.
View Article PubMed Google Scholar -
Grogan
S.P.,
D'Lima
D.D.,
Joint aging and chondrocyte cell death. International Journal of Clinical Rheumatology.
2010;
5
(2)
:
199-214
.
View Article PubMed Google Scholar -
Knudson
C.B.,
Knudson
W.,
Cartilage proteoglycans. Seminars in Cell & Developmental Biology.
2001;
12
(2)
:
69-78
.
View Article PubMed Google Scholar -
Hunziker
E.B.,
Quinn
T.M.,
Hauselmann
H.J.,
Quantitative structural organization of normal adult human articular cartilage. Osteoarthritis Cartilage.
2002;
10
(7)
:
564-567
.
View Article PubMed Google Scholar -
Roughley
P.J.,
The structure and function of cartilage proteoglycans. European Cells & Materials.
2006;
12
:
92-101
.
View Article PubMed Google Scholar -
Williams
G.M.,
Klisch
S.M.,
Sah
R.L.,
Bioengineering cartilage growth, maturation, and form. Pediatric Research.
2008;
63
(5)
:
527-34
.
View Article PubMed Google Scholar -
Maldonado
M.,
Nam
J.,
The role of changes in extracellular matrix of cartilage in the presence of inflammation on the pathology of osteoarthritis. BioMed Research International.
2013;
2013
:
284873
.
View Article PubMed Google Scholar -
Martino
F.,
Perestrelo
A.R.,
Vinarský
V.,
Pagliari
S.,
Forte
G.,
Cellular Mechanotransduction: From Tension to Function. Frontiers in Physiology.
2018;
9
:
824
.
View Article PubMed Google Scholar -
Trengove
A.,
Di Bella
C.,
O'Connor
A.J.,
The Challenge of Cartilage Integration: Understanding a Major Barrier to Chondral Repair. Tissue Engineering. Part B, Reviews.
2022;
28
(1)
:
114-28
.
View Article PubMed Google Scholar -
Fan
D.,
Wang
Q.,
Zhu
T.,
Wang
H.,
Liu
B.,
Wang
Y.,
Recent Advances of Magnetic Nanomaterials in Bone Tissue Repair. Frontiers in Chemistry.
2020;
8
:
745
.
View Article PubMed Google Scholar -
Stanicki
D.,
Elst
L.V.,
Muller
R.N.,
Laurent
S.,
Synthesis and processing of magnetic nanoparticles. Current Opinion in Chemical Engineering.
2015;
8
:
7-14
.
View Article Google Scholar -
Qin
J.,
Laurent
S.,
Jo
Y.S.,
Roch
A.,
Mikhaylova
M.,
Bhujwalla
Z.M.,
A high-performance magnetic resonance imaging T2 contrast agent. Advanced Materials.
2007;
19
(14)
:
1874-8
.
View Article Google Scholar -
Issa
B.,
Obaidat
I.M.,
Albiss
B.A.,
Haik
Y.,
Magnetic nanoparticles: surface effects and properties related to biomedicine applications. International Journal of Molecular Sciences.
2013;
14
(11)
:
21266-305
.
View Article PubMed Google Scholar -
Mahshid
S.S.,
Mahshid
S.,
Dolati
A.,
Ghorbani
M.,
Yang
L.,
Luo
S.,
Electrodeposition and electrocatalytic properties of Pt/Ni–Co nanowires for non-enzymatic glucose detection. Journal of Alloys and Compounds.
2013;
554
:
169-76
.
View Article Google Scholar -
Markides
H.,
Rotherham
M.,
ElHaj
A.J.,
Biocompatibility and Toxicity of Magnetic Nanoparticles in Regenerative Medicine Journal of Nanomaterials. Journal of Nanomaterials.
2012;
2012
:
614094
.
View Article Google Scholar -
Mahmoudi
M.,
Hofmann
H.,
Rothen-Rutishauser
B.,
Petri-Fink
A.,
Assessing the in vitro and in vivo toxicity of superparamagnetic iron oxide nanoparticles. Chemical Reviews.
2012;
112
(4)
:
2323-38
.
View Article PubMed Google Scholar -
Nicewarner-Pena
S.R.,
Freeman
R.G.,
Reiss
B.D.,
He
L.,
Pena
D.J.,
Walton
I.D.,
Submicrometer metallic barcodes. Science.
2001;
294
(5540)
:
137-41
.
View Article PubMed Google Scholar -
Choi
D.,
Fung
A.,
Moon
H.,
Ho
D.,
Chen
Y.,
Kan
E.,
Transport of living cells with magnetically assembled nanowires. Biomedical Microdevices.
2007;
9
(2)
:
143-8
.
View Article PubMed Google Scholar -
Labusca
L.,
Danceanu
C.,
Minuti
A.E.,
Herea
D.D.,
Ghemes
A.,
Rotarescu
C.,
Magnetic nanowires substrate increases adipose-derived mesenchymal cells osteogenesis. Scientific Reports.
2022;
12
(1)
:
16698
.
View Article PubMed Google Scholar -
Tiberto
P.,
Barrera
G.,
Celegato
F.,
Conta
G.,
Coisson
M.,
Vinai
F.,
Ni80Fe20 nanodisks by nanosphere lithography for biomedical applications. Journal of Applied Physics.
2015;
117
:
17B304
.
View Article Google Scholar -
Labusca
L.,
Herea
D.-D.,
Dragos-Pinzaru
O.,
Chiriac
H.,
Lupu
N.,
Magnetic Nanoparticles in Regenerative Medicine - Current Role and Future Perspectives. Frontiers in Stem Cell and Regenerative Medicine Research.
2017;
7
:
163
.
View Article Google Scholar -
Wei
Y.,
Zhao
M.,
Yang
F.,
Mao
Y.,
Xie
H.,
Zhou
Q.,
Iron overload by Superparamagnetic Iron Oxide Nanoparticles is a High Risk Factor in Cirrhosis by a Systems Toxicology Assessment. Scientific Reports.
2016;
6
(1)
:
29110
.
View Article PubMed Google Scholar -
Adedoyin
A.A.,
Ekenseair
A.K.,
Biomedical applications of magneto-responsive scaffolds. Nano Research.
2018;
11
(10)
:
5049-64
.
View Article Google Scholar -
Brunger
J.M.,
Huynh
N.P.,
Guenther
C.M.,
Perez-Pinera
P.,
Moutos
F.T.,
Sanchez-Adams
J.,
Scaffold-mediated lentiviral transduction for functional tissue engineering of cartilage. Proceedings of the National Academy of Sciences of the United States of America.
2014;
111
(9)
:
798-806
.
View Article PubMed Google Scholar -
Zhang
N.,
Lock
J.,
Sallee
A.,
Liu
H.,
Magnetic nanocomposite hydrogel for potential cartilage tissue engineering: synthesis, characterization, and cytocompatibility with bone marrow derived mesenchymal stem cells. ACS Applied Materials & Interfaces.
2015;
7
(37)
:
20987-98
.
View Article PubMed Google Scholar -
Hou
R.,
Nie
L.,
Du
G.,
Xiong
X.,
Fu
J.,
Natural polysaccharides promote chondrocyte adhesion and proliferation on magnetic nanoparticle/PVA composite hydrogels. Colloids and Surfaces. B, Biointerfaces.
2015;
132
:
146-54
.
View Article PubMed Google Scholar -
Yang
W.,
Zhu
P.,
Huang
H.,
Zheng
Y.,
Liu
J.,
Feng
L.,
Functionalization of Novel Theranostic Hydrogels with Kartogenin-Grafted USPIO Nanoparticles To Enhance Cartilage Regeneration. ACS Applied Materials & Interfaces.
2019;
11
(38)
:
34744-54
.
View Article PubMed Google Scholar -
Tanaka
H.,
Sugita
T.,
Yasunaga
Y.,
Shimose
S.,
Deie
M.,
Kubo
T.,
Efficiency of magnetic liposomal transforming growth factor-beta 1 in the repair of articular cartilage defects in a rabbit model. Journal of Biomedical Materials Research. Part A.
2005;
73
(3)
:
255-63
.
View Article PubMed Google Scholar -
Zhang
C.,
Cai
Y.Z.,
Lin
X.J.,
Wang
Y.,
Magnetically Actuated Manipulation and Its Applications for Cartilage Defects: Characteristics and Advanced Therapeutic Strategies. Frontiers in Cell and Developmental Biology.
2020;
8
:
526
.
View Article PubMed Google Scholar -
Kim
J.,
Tanner
K.,
Three-Dimensional Patterning of the ECM Microenvironment Using Magnetic Nanoparticle Self Assembly. Current protocols in cell biology.
2016;
70
:
25.3.1-25.3.14
.
View Article PubMed Google Scholar -
Margolis
G.,
Polyak
B.,
Cohen
S.,
Magnetic Induction of Multiscale Anisotropy in Macroporous Alginate Scaffolds. Nano Letters.
2018;
18
(11)
:
7314-22
.
View Article PubMed Google Scholar -
Xu
J.,
Feng
Q.,
Lin
S.,
Yuan
W.,
Li
R.,
Li
J.,
Injectable stem cell-laden supramolecular hydrogels enhance in situ osteochondral regeneration via the sustained co-delivery of hydrophilic and hydrophobic chondrogenic molecules. Biomaterials.
2019;
210
:
51-61
.
View Article PubMed Google Scholar -
Brady
M.A.,
Talvard
L.,
Vella
A.,
Ethier
C.R.,
Bio-inspired design of a magnetically active trilayered scaffold for cartilage tissue engineering. Journal of Tissue Engineering and Regenerative Medicine.
2017;
11
(4)
:
1298-302
.
View Article PubMed Google Scholar -
Arias
S.L.,
Shetty
A.,
Devorkin
J.,
Allain
J.P.,
Magnetic targeting of smooth muscle cells in vitro using a magnetic bacterial cellulose to improve cell retention in tissue-engineering vascular grafts. Acta Biomaterialia.
2018;
77
:
172-81
.
View Article PubMed Google Scholar -
Wong
D.S.,
Li
J.,
Yan
X.,
Wang
B.,
Li
R.,
Zhang
L.,
Magnetically Tuning Tether Mobility of Integrin Ligand Regulates Adhesion, Spreading, and Differentiation of Stem Cells. Nano Letters.
2017;
17
(3)
:
1685-95
.
View Article PubMed Google Scholar -
Zhao
Y.,
Fan
T.,
Chen
J.,
Su
J.,
Zhi
X.,
Pan
P.,
Magnetic bioinspired micro/nanostructured composite scaffold for bone regeneration. Colloids and Surfaces. B, Biointerfaces.
2019;
174
:
70-9
.
View Article PubMed Google Scholar -
Sensenig
R.,
Sapir
Y.,
MacDonald
C.,
Cohen
S.,
Polyak
B.,
Magnetic nanoparticle-based approaches to locally target therapy and enhance tissue regeneration in vivo. Nanomedicine (London).
2012;
7
(9)
:
1425-42
.
View Article PubMed Google Scholar -
Thevenot
P.,
Sohaebuddin
S.,
Poudyal
N.,
Liu
J.P.,
Tang
L.,
Magnetic Nanoparticles to Enhance Cell Seeding and Distribution in Tissue Engineering Scaffolds. Proceedings of the IEEE Conference on Nanotechnology.
2008;
2008
:
646-9
.
View Article PubMed Google Scholar -
Ito
A.,
Takizawa
Y.,
Honda
H.,
Hata
K.,
Kagami
H.,
Ueda
M.,
Tissue engineering using magnetite nanoparticles and magnetic force: heterotypic layers of cocultured hepatocytes and endothelial cells. Tissue Engineering.
2004;
10
(5-6)
:
833-40
.
View Article PubMed Google Scholar -
Shimizu
K.,
Ito
A.,
Yoshida
T.,
Yamada
Y.,
Ueda
M.,
Honda
H.,
Bone tissue engineering with human mesenchymal stem cell sheets constructed using magnetite nanoparticles and magnetic force. Journal of Biomedical Materials Research. Part B, Applied Biomaterials.
2007;
82
(2)
:
471-80
.
View Article PubMed Google Scholar -
Luciani
N.,
Du
V.,
Gazeau
F.,
Richert
A.,
Letourneur
D.,
Le Visage
C.,
Successful chondrogenesis within scaffolds, using magnetic stem cell confinement and bioreactor maturation. Acta Biomaterialia.
2016;
37
:
101-10
.
View Article PubMed Google Scholar -
Fayol
D.,
Frasca
G.,
Le Visage
C.,
Gazeau
F.,
Luciani
N.,
Wilhelm
C.,
Use of magnetic forces to promote stem cell aggregation during differentiation, and cartilage tissue modeling. Advanced Materials.
2013;
25
(18)
:
2611-6
.
View Article PubMed Google Scholar -
Mahmoud
E.E.,
Kamei
G.,
Harada
Y.,
Shimizu
R.,
Kamei
N.,
Adachi
N.,
Cell Magnetic Targeting System for Repair of Severe Chronic Osteochondral Defect in a Rabbit Model. Cell Transplantation.
2016;
25
(6)
:
1073-83
.
View Article PubMed Google Scholar -
Kamei
G.,
Kobayashi
T.,
Ohkawa
S.,
Kongcharoensombat
W.,
Adachi
N.,
Takazawa
K.,
Articular cartilage repair with magnetic mesenchymal stem cells. The American Journal of Sports Medicine.
2013;
41
(6)
:
1255-64
.
View Article PubMed Google Scholar -
Hori
J.,
Deie
M.,
Kobayashi
T.,
Yasunaga
Y.,
Kawamata
S.,
Ochi
M.,
Articular cartilage repair using an intra-articular magnet and synovium-derived cells. Journal of Orthopaedic Research.
2011;
29
(4)
:
531-8
.
View Article PubMed Google Scholar -
Vidiasheva
I.V.,
Abalymov
A.A.,
Kurochkin
M.A.,
Mayorova
O.A.,
Lomova
M.V.,
German
S.V.,
Transfer of cells with uptaken nanocomposite, magnetite-nanoparticle functionalized capsules with electromagnetic tweezers. Biomaterials Science.
2018;
6
(8)
:
2219-29
.
View Article PubMed Google Scholar -
Bayoussef
Z.,
Dixon
J.E.,
Stolnik
S.,
Shakesheff
K.M.,
Aggregation promotes cell viability, proliferation, and differentiation in an in vitro model of injection cell therapy. Journal of Tissue Engineering and Regenerative Medicine.
2012;
6
(10)
:
e61-73
.
View Article PubMed Google Scholar -
Ghosh
S.,
Kumar
S.R.,
Puri
I.K.,
Elankumaran
S.,
Magnetic assembly of 3D cell clusters: visualizing the formation of an engineered tissue. Cell Proliferation.
2016;
49
(1)
:
134-44
.
View Article PubMed Google Scholar -
Souza
G.R.,
Molina
J.R.,
Raphael
R.M.,
Ozawa
M.G.,
Stark
D.J.,
Levin
C.S.,
Three-dimensional tissue culture based on magnetic cell levitation. Nature Nanotechnology.
2010;
5
(4)
:
291-6
.
View Article PubMed Google Scholar -
V.T. Tu,
H.T. Le,
X.H. To,
P.D. Nguyen,
P.D. Huynh,
T.M. Le,
N.B. Vu,
Method for in vitro production of cartilage microtissues from scaffold-free spheroids composed of human adipose-derived stem cells. Biomedical Research and Therapy.
2020;
7
(4)
:
3697-708
.
View Article Google Scholar -
Labusca
L.,
Herea
D.D.,
Minuti
A.E.,
Stavila
C.,
Danceanu
C.,
Grigoras
M.,
Magnetic nanoparticle loaded human adipose derived mesenchymal cells spheroids in levitated culture. Journal of Biomedical Materials Research. Part B, Applied Biomaterials.
2021;
109
(5)
:
630-42
.
View Article PubMed Google Scholar -
Koto
W.,
Shinohara
Y.,
Kitamura
K.,
Wachi
T.,
Makihira
S.,
Koyano
K.,
Porcine Dental Epithelial Cells Differentiated in a Cell Sheet Constructed by Magnetic Nanotechnology. Nanomaterials (Basel, Switzerland).
2017;
7
(10)
:
322
.
View Article PubMed Google Scholar -
Ishii
M.,
Shibata
R.,
Numaguchi
Y.,
Kito
T.,
Suzuki
H.,
Shimizu
K.,
Enhanced angiogenesis by transplantation of mesenchymal stem cell sheet created by a novel magnetic tissue engineering method. Arteriosclerosis, Thrombosis, and Vascular Biology.
2011;
31
(10)
:
2210-5
.
View Article PubMed Google Scholar -
Vinhas
A.,
Gonçalves
A.I.,
Rodrigues
M.T.,
Gomes
M.E.,
Human tendon-derived cell sheets created by magnetic force-based tissue engineering hold tenogenic and immunomodulatory potential. Acta Biomaterialia.
2021;
131
:
236-47
.
View Article PubMed Google Scholar -
Ito
S.,
Sato
M.,
Yamato
M.,
Mitani
G.,
Kutsuna
T.,
Nagai
T.,
Repair of articular cartilage defect with layered chondrocyte sheets and cultured synovial cells. Biomaterials.
2012;
33
(21)
:
5278-86
.
View Article PubMed Google Scholar -
Zhang
W.,
Yang
G.,
Wang
X.,
Jiang
L.,
Jiang
F.,
Li
G.,
Magnetically Controlled Growth-Factor-Immobilized Multilayer Cell Sheets for Complex Tissue Regeneration. Advanced Materials.
2017;
29
(43)
:
1703795
.
View Article PubMed Google Scholar -
Su
J.Y.,
Chen
S.H.,
Chen
Y.P.,
Chen
W.C.,
Evaluation of Magnetic Nanoparticle-Labeled Chondrocytes Cultivated on a Type II Collagen-Chitosan/Poly(Lactic-co-Glycolic) Acid Biphasic Scaffold. International Journal of Molecular Sciences.
2017;
18
(1)
:
87
.
View Article PubMed Google Scholar -
Van de Walle
A.,
Plan Sangnier
A.,
Abou-Hassan
A.,
Curcio
A.,
Hémadi
M.,
Menguy
N.,
Biosynthesis of magnetic nanoparticles from nano-degradation products revealed in human stem cells. Proceedings of the National Academy of Sciences of the United States of America.
2019;
116
(10)
:
4044-53
.
View Article PubMed Google Scholar -
Liu
X.,
Liu
J.,
Kang
N.,
Yan
L.,
Wang
Q.,
Fu
X.,
Role of insulin-transferrin-selenium in auricular chondrocyte proliferation and engineered cartilage formation in vitro. International Journal of Molecular Sciences.
2014;
15
(1)
:
1525-37
.
View Article PubMed Google Scholar -
Mehta
K.J.,
Role of iron and iron-related proteins in mesenchymal stem cells: cellular and clinical aspects. Journal of Cellular Physiology.
2021;
236
(10)
:
7266-89
.
View Article PubMed Google Scholar -
Theruvath
A.J.,
Mahmoud
E.E.,
Wu
W.,
Nejadnik
H.,
Kiru
L.,
Liang
T.,
Ascorbic Acid and Iron Supplement Treatment Improves Stem Cell-Mediated Cartilage Regeneration in a Minipig Model. The American Journal of Sports Medicine.
2021;
49
(7)
:
1861-70
.
View Article PubMed Google Scholar -
Labusca
L.,
Herea
D.D.,
Emanuela Minuti
A.,
Stavila
C.,
Danceanu
C.,
Plamadeala
P.,
Magnetic Nanoparticles and Magnetic Field Exposure Enhances Chondrogenesis of Human Adipose Derived Mesenchymal Stem Cells But Not of Wharton Jelly Mesenchymal Stem Cells. Frontiers in Bioengineering and Biotechnology.
2021;
9
:
737132
.
View Article PubMed Google Scholar -
Huang
J.,
Xiong
J.,
Wang
D.,
Zhang
J.,
Yang
L.,
Sun
S.,
3D Bioprinting of Hydrogels for Cartilage Tissue Engineering. Gels (Basel, Switzerland).
2021;
7
(3)
:
144
.
View Article PubMed Google Scholar -
Bonhome-Espinosa
A.B.,
Campos
F.,
Durand-Herrera
D.,
Sánchez-López
J.D.,
Schaub
S.,
Durán
J.D.,
In vitro characterization of a novel magnetic fibrin-agarose hydrogel for cartilage tissue engineering. Journal of the Mechanical Behavior of Biomedical Materials.
2020;
104
:
103619
.
View Article PubMed Google Scholar -
Simińska-Stanny
J.,
Nizioł
M.,
Szymczyk-Ziółkowska
P.,
Brożyna
M.,
Junka
A.,
Shavandi
A.,
4D printing of patterned multimaterial magnetic hydrogel actuators. Additive Manufacturing.
2022;
49
:
102506
.
View Article Google Scholar -
Zlotnick
H.M.,
Clark
A.T.,
Gullbrand
S.E.,
Carey
J.L.,
Cheng
X.M.,
Mauck
R.L.,
Magneto-Driven Gradients of Diamagnetic pubs for Engineering Complex Tissues. Advanced Materials.
2020;
32
(48)
:
e2005030
.
View Article PubMed Google Scholar -
L.J. Santos,
R.L. Reis,
M.E. Gomes,
Harnessing magnetic-mechano actuation in regenerative medicine and tissue engineering. Trends in biotechnology.
2015;
33
(8)
:
471-479
.
View Article Google Scholar
Comments
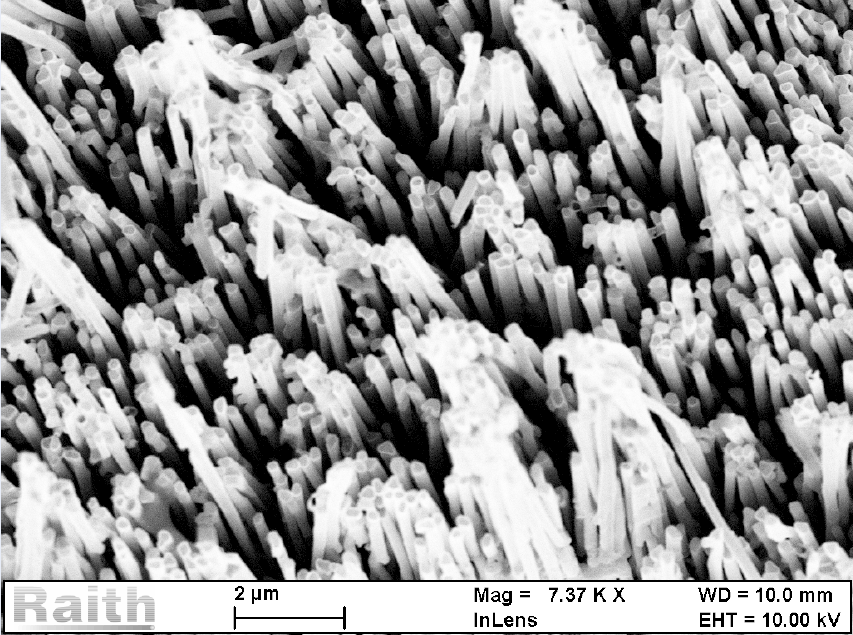
Article Details
Volume & Issue : Vol 10 No 1 (2023)
Page No.: 5509-5522
Published on: 2023-01-31
Citations
Copyrights & License

This work is licensed under a Creative Commons Attribution 4.0 International License.
Search Panel
- HTML viewed - 4082 times
- PDF downloaded - 1108 times
- XML downloaded - 0 times