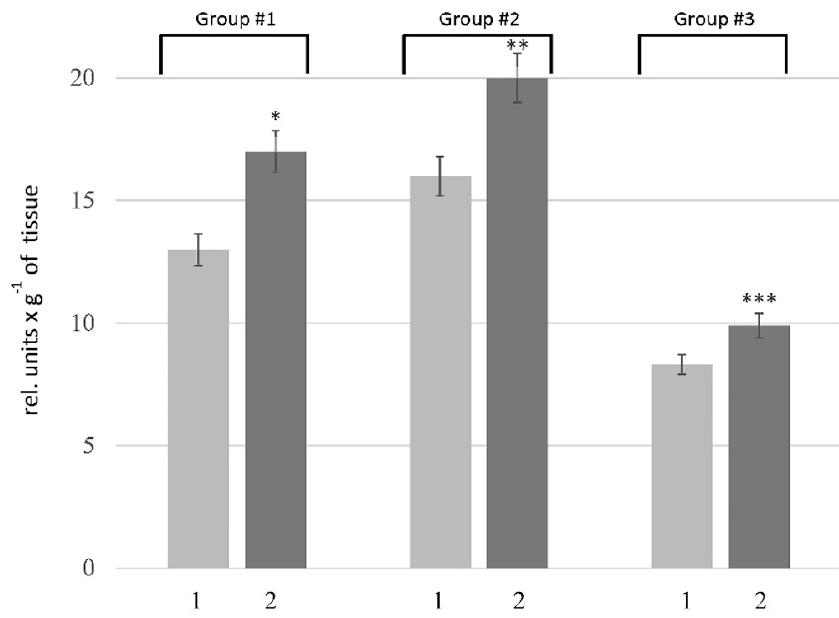
Disorders of proteolytic homeostasis in the liver of rats with hyperhomocysteinemia
- Educational and Scientific Centre “Institute of Biology and Medicine” Taras Shevchenko National University of Kyiv, Volodymyrska 64/13, 01601, Kyiv, Ukraine
- National Pirogov Memorial Medical University of Vinnytsya, Pirogova 56, 21018, Vinnytsya, Ukraine
Abstract
Introduction: The liver is a key organ of the body that is responsible for maintaining homeostasis. It helps regulate almost all biochemical pathways associated with metabolism, nutrition, energy supply, and the formation of immunity. Consequently, impaired liver function can contribute to the progression of hyperhomocysteinemia and the development of associated complications. The present study aims to investigate the level and activity of metal-dependent and serine proteases in the liver of rats with hyperhomocysteinemia.
Methods: A total of 60 albino nonlinear male rats were used in this study. Hyperhomocysteinemia was induced by intragastric administration of DL-homocysteine thiolactone. Total proteolytic activity was measured using casein as a substrate. To determine the activity of metal-dependent and serine proteases, ethylenediaminetetraacetic acid and phenylmethylsulfonyl fluoride were used. The levels of MMP-1, MMP-2, and MMP-3 were studied by enzyme-linked immunosorbent assay. The fraction of serine proteases was isolated by affinity chromatography on the benzamidine sepharose column. The composition of serine protease fraction was analyzed by sodium dodecyl sulfate-polyacrylamide gel electrophoresis.
Results: The pathogenesis of hyperhomocysteinemia was found to be accompanied by a proteolytic imbalance manifested in the increase in the total proteolytic activity, the activity of serine proteases and especially the activity of metal-dependent enzymes. The increase in the levels of MMP-1, MMP-2, and MMP-3 indicates the involvement of these enzymes in the amplification of proteolysis mediated by metal-dependent enzymes. Despite a significant increase in the content of the serine proteases, their activity did not increase significantly. This may be because of the proteolytic degradation of serine proteases, which was confirmed by changes in their qualitative composition. The accumulation of low-molecular-weight proteins and the decrease in the part of proteins with a molecular weight of greater than 50 kDa in the fraction of serine proteases were revealed.
Conclusion: Collectively, our observations indicate that impaired proteolysis in the liver may be an important determinant in the development of hyperhomocysteinemia-associated disorders.
Introduction
Hyperhomocysteinemia (HM) is considered to be a condition characterized by an abnormally high level of homocysteine1 in the blood1. It has been established that an elevated level of homocysteine is an independent risk factor for cardiovascular diseases, neurodegenerative diseases, liver disorders, and kidney disorders2, 3. Although the primary cause of HM is defects in the genes involved in homocysteine metabolism, the prevalence of this disease is now growing rapidly. HM can develop as a consequence of nutritional conditions such as a deficiency of vitamins B6 and B12 or folic acid, hazardous habits, such as chronic alcohol consumption or smoking, and taking certain medications, such as cholestyramine and metformin4, 5.
The liver is a key organ of the body responsible for maintaining homeostasis. It is involved in regulating almost all biochemical pathways associated with metabolism, nutrition, energy supply, and the formation of immunity6. The liver is the most prominent site for the synthesis of enzymes that contribute to homocysteine metabolism. Consequently, impaired liver function can contribute to the further progression of HM and the development of associated complications. A better understanding of the pathogenetic mechanisms of homocysteine-induced changes in the liver is critical for the proper and effective treatment of HM and, if possible, to prevent the development of certain severe complications such as liver fibrosis, cirrhosis, or even cancer. In this work, we investigated proteolytic homeostasis, especially the activity and level of serine and metal-dependent proteases in the liver of HM rats.
Methods
Reagents
Thiolactone D,L-homocysteine, tris(hydroxymethyl)aminomethane, ethylenediaminetetraacetic acid, phenylmethylsulfonyl fluoride, o-phenylenediamine, hydrogen peroxide, sodium dodecyl sulfate, Coomassie Blue R-250, acrylamide, and N,N'-methylenebisacrylamide were purchased from Sigma-Aldrich (St. Louis, MO, USA). Anti-rat monoclonal antibodies to INF-, IL-1, IL-4, IL-10, IL-12, MMP-1, MMP-2, and MMP-3 were purchased from Santa Cruz Biotechnology, Inc. (Dallas, Texas, USA). Horseradish peroxidase-conjugated secondary antibodies were purchased from Sigma-Aldrich (St. Louis, MO, USA). All other chemicals and reagents used in this study were of analytical grade quality and available commercially.
Animals and experimental design
A total of 60 albino nonlinear male rats were used in this study. All experiments on animals were performed in compliance with the international principles of the European Convention for the protection of vertebrate animals used for experimental and other scientific purposes (Strasbourg, 1986). The study was approved by the Ethical Committee of Taras Shevchenko National University of Kyiv. The experiments started after 7 days of animal acclimation in the animal facility of Taras Shevchenko National University of Kyiv, maintained under constant conditions of temperature (22 ± 3°C), humidity (60 ± 5%), and light (12 h light/12 h dark cycle). Standard rodent food and water were provided ad libitum. The animals of different ages were used in the current study: one-month-old rats that are corresponded to young animals, six-month-old rats that are corresponded to adult animals, and twenty-month-old rats that are corresponded to old animals. HM was induced by intragastric administration of thiolactone D,L-homocysteine diluted in 1% starch solution (100 mg·kg of body weight), once per day for 28 days. The control rats received an equal volume of 1% starch. The development of HM was confirmed by the high blood level of homocysteine (>15 mmol·L). On the 29 day of the experiment, animals were sacrificed. Thus, there were three experimental groups that included young, adult, and old animals7with HM: Group #1 (rats aged 1 – 2 months; n = 10), Group #2 (rats aged 6 – 8 months; n = 10), and Group #3 (rats aged 24 – 26 months; n = 10). The control groups (n = 10) consisted of animals of the same age as in the corresponding experimental group.
Liver sample preparation
The liver was collected immediately after the animals were sacrificed. The tissue (1 g) was homogenized in 9 mL ice-cold 50 mM Tris–HCl buffer (pH 7.4). The homogenate solution was centrifuged at 12,000 g for 30 min at 4°C. The supernatant was collected and stored at 80°C for further analysis. The protein concentration was determined by the Bradford method using crystalline bovine serum albumin as a standard.
Determination of total proteolytic activity,theactivity of metal-dependent and serine proteases
The total proteolytic activity was measured using casein as a substrate according to the method8. Casein (2%) in 50 mM Tris–HCl buffer (pH 7.4) containing 0.13 M NaCl was incubated in the presence of a tested sample (50 µg of total protein) at 37 °C for 30 min. The reaction was stopped by the addition of trichloroacetic acid (7%) and the sample was allowed to stand for 15 min at 4 °C. The mixture was centrifuged at 15,000 g for 30 min. The absorbance of the supernatant was measured spectrophotometrically (SmartSpec Plus, Bio-Rad, USA) at 280 nm against the blank in which the tested sample was substituted by a corresponding volume of 50 mM Tris–HCl buffer (pH 7.4) containing 0.13 M NaCl. Total proteolytic activity was expressed as rel. units·g of liver tissue.
The activities of metal-dependent and serine proteases were studied using corresponding inhibitors: ethylenediaminetetraacetic acid for the estimation of metal-dependent enzymes and phenylmethylsulfonyl fluoride for the estimation of serine proteases. Both inhibitors were used at the final concentration of 5 mM. The tested samples were pre-incubated with an inhibitor for 30 min at 4°C, and then the remaining enzyme activity was estimated using casein as a substrate.
Isolation of the fraction of serine proteases
The fraction of serine proteases was isolated by affinity chromatography on the Benzamidine Sepharose4 Fast Flow (GE Healthcare, USA)9. The liver samples were loaded to the column that was pre-equilibrated with 10 mM Tris–HCl (pH 8.0). Elution of the adsorbed proteins was carried out using a 50 mM glycine–HCl buffer at pH 3.0 containing 1 M NaCl. The sample was loaded at a flow rate of 60 mL per hour, and the fraction of serine proteases was collected at a flow rate of 120 mL per hour. The concentration of serine proteases was determined using the Bradford method10, and then the obtained value was converted to gram of liver tissue.
Determination of the levels of MMPs
The levels of MMPs were measured by enzyme-linked immunosorbent assay (ELISA) according to the standard instructions11. ELISA plates were coated overnight at 4°C with samples of heart homogenate previously diluted with Tris–HCl buffer (pH 7.4) to obtain the concentration of proteins of 10 μg·mL. After being washed, the plates were capped with 5% nonfat dry milk for 1 h at 37°C and washed again. After that, the plates were incubated for 1 h at 37°C with specific primary antibodies against the MMP-1, MMP-2, and MMP-3. Plates were washed and incubated for 1 h at 37°C with corresponding secondary antibodies conjugated to horseradish peroxidase. After washing, substrate (o-phenylenediamine and hydrogen peroxide) was added. The reaction was stopped by the addition of 2.5 N HSO. The plates were read at 492 nm by a microplate reader (mQuant™, BioTek Instruments, Inc.).
Sodium dodecyl sulfate-polyacrylamide gel electrophoresis (SDS-PAGE)
SDS-PAGE was performed as reported by Laemmli12, using 4% (w/v) stacking gel and 10% (w/v) resolving gel. The samples were prepared by mixing with the sample buffer (0.05 M Tris–HCl, pH 8.8, 2% SDS, 5% sucrose, and 0.02% bromophenol blue) at a ratio of 1:1 (v/v). The samples were heated at 95ºC for 1 min prior to loading in the gel. The total amount of proteins applied per well of gel was 20 µg. Electrophoresis was performed at 39 mA per resolving gel in Tris–glycine buffer, pH 8.3, containing 0.01% SDS. Gels were stained with Coomassie Blue R-250. The molecular weights of proteins were estimated using a low-molecular-weight calibration kit (Bio-Rad, USA).
Statistical analysis
The data of biochemical estimations were reported as the mean ± SEM for each group (n = 10). Statistical analyses were performed using a one-way analysis of variance (ANOVA). Differences were considered to be statistically significant when p < 0.05.
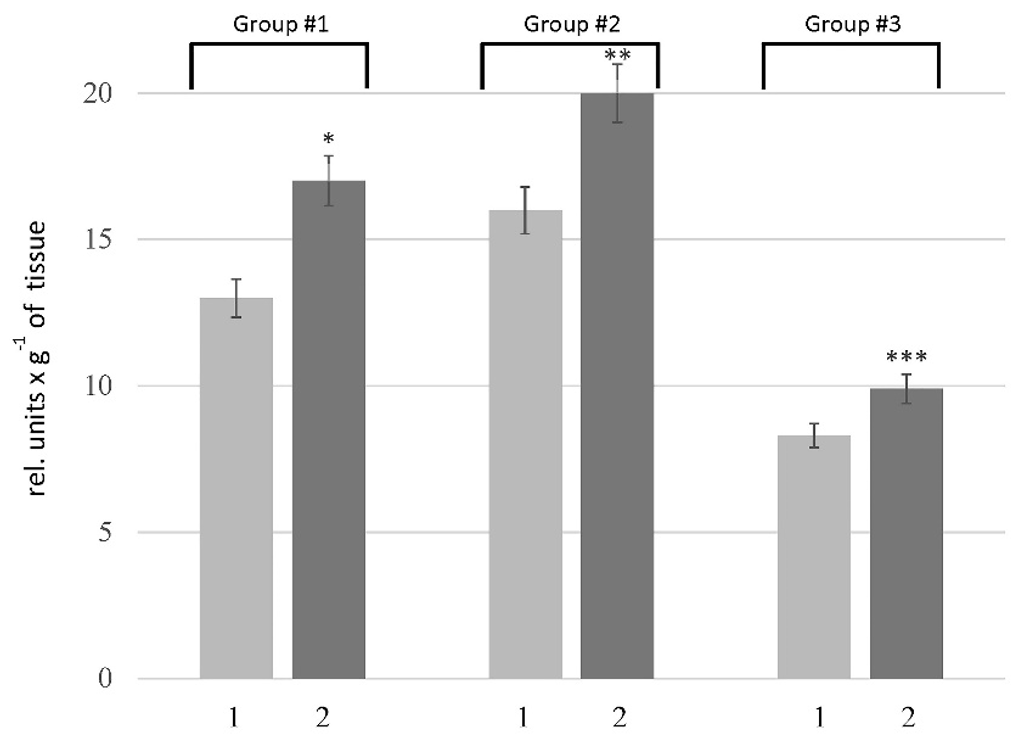
Total proteolytic activity in the liver of rats: 1 — the control rats; 2 — the rats with HM. Values are expressed as mean ± SEM (n = 10); *p < 0.05 significantly different from the control of Group # 1; **p < 0.05 significantly different from the control of Group # 2; ***p < 0.05 significantly different from the control of Group # 3
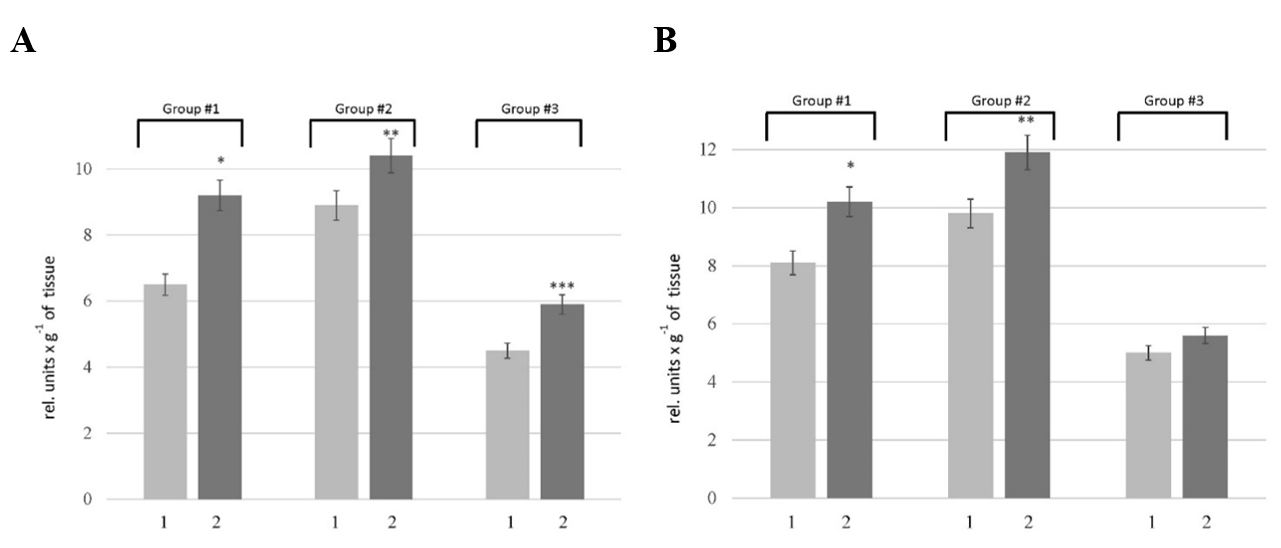
Activity of metal-dependent (A) and serine proteases (B) in the liver of rats: 1 — the control rats; 2 — the rats with HM. Values are expressed as mean ± SEM (n = 10); *p < 0.05 significantly different from the control of Group # 1; **p < 0.05 significantly different from the control of Group # 2; ***p < 0.05 significantly different from the control of Group # 3
Results of qualitative analysis of serine proteinase fraction
|
Group # 1 |
Group # 2 |
Group # 3 | |||
Protein fraction (kDa) |
Control (%) |
HM (%) |
Control (%) |
HM (%) |
Control (%) |
HM (%) |
> 150 |
- |
- |
- |
- |
- |
- |
150 - 100 |
36.61 ± 1.87 |
8.32 ± 0.41 * |
25.57 ± 1.23 |
8.23 ± 0.40 ** |
38.28 ± 1.87 |
3.67 ± 0.18 *** |
100 - 70 |
29.56 ± 1.35 |
7.75 ± 0.35 * |
25.48 ± 1.24 |
6.13 ± 0.30 ** |
25.31 ± 1.25 |
4.76 ± 0.21 *** |
70 - 50 |
20.43 ± 1.01 |
8.03 ± 0.38 * |
23.47 ± 1.20 |
2.62 ± 0.11 ** |
21.46 ± 1.03 |
16.0 ± 0.80 *** |
50 - 30 |
11.82 ± 0.54 |
22.18 ± 1.09 * |
14.14 ± 0.07 |
12.45 ± 0.57 ** |
5.94 ± 0.25 |
1.46 ± 0.07 *** |
˂ 30 |
1.61 ± 0.08 |
53.70 ± 2.63 * |
11.33 ± 0.47 |
70.56 ± 3.51 ** |
9.02 ± 0.41 |
74.12 ± 3.51 *** |
Level of MMPs in the liver of rats with hyperhomocysteinemia
|
Group #1 |
Group #2 |
Group #3 | |||
rel. units·mg-1 of proteins | ||||||
Control |
HM |
Control |
HM |
Control |
HM | |
MMP-1 |
17.10 ± 0.85 |
40.21 ± 2.1 * |
43.26 ± 2.15 |
35.42 ± 1.65 ** |
49.75 ± 2.41 |
57.85 ± 2.85 *** |
MMP-2 |
62.35 ± 3.12 |
80.00 ± 3.85 * |
70.23 ± 3.44 |
141.13 ± 7.00 ** |
75.37 ± 3.75 |
145.21 ± 7.15 *** |
MMP-3 |
99.77 ± 4.55 |
147.31 ± 7.25 * |
81.04 ± 4.05 |
121.43 ± 6.00 ** |
73.41 ± 3.65 |
95.22 ± 4.75 *** |
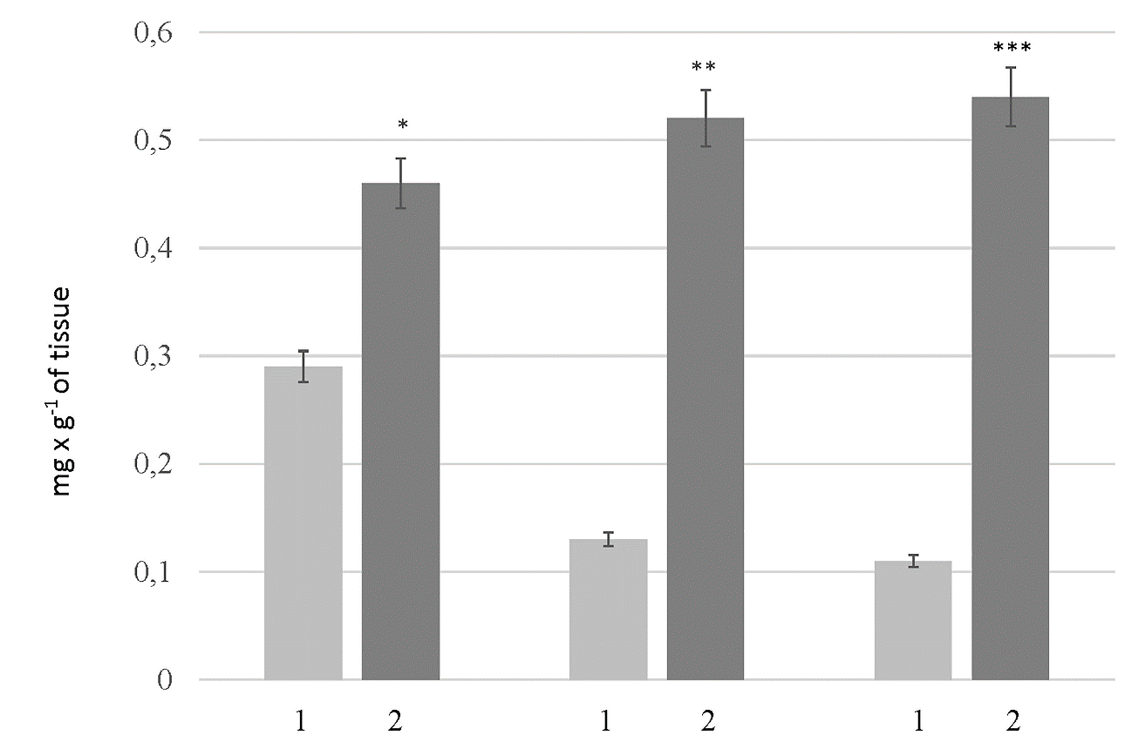
Level of serine proteases in the liver of rats: 1 — the control rats; 2 — the rats with HM. Values are expressed as mean ± SEM (n = 10); *p < 0.05 significantly different from the control of Group # 1; **p<0.05 significantly different from the control of Group #2; ***p < 0.05 significantly different from the control of Group # 3.
Results
Total proteolytic activity, the activity of metal-dependent and serine proteases
The proteolytic activity in the liver was determined using casein as a substrate, which allows assessing the overall proteolytic activity. Our results showed an increase in this parameter in the rats of all tested groups. As depicted in Figure 1, the pathogenesis of HM was accompanied by 1.3-, 1.2-, and 1.1-fold increase in the caseinolytic activity in the liver of rats of Groups # 1, # 2, and # 3, respectively. To clarify the nature of the primary enzymes responsible for enhancing proteolysis in the liver of rats with HM, the activity of metal-dependent and serine proteases was estimated. According to the data (Figure 2A), an increase in the activity of metal-dependent enzymes was found in HM rats. The activity of metal-dependent enzymes was higher than those in the corresponding controls by 42% in the rats of Group # 1, 17% in the rats of Group # 2, and 31% in the rats of Group #3. We also revealed the increase in the activity of serine proteases (Figure 2B). However, the alterations of serine protease activity were not as pronounced as in the case of metal-dependent enzymes. The activity of serine proteases in the rats of Group # 1 and # 2 was increased by 12 — 23% compared with corresponding controls. No changes in this parameter were found in the liver of rats of Group # 3.
Level and qualitative composition of serine proteases
To analyze the qualitative composition of serine proteases in the liver of HM rats, the fraction of serine proteases was isolated. The fraction was isolated by affinity chromatography on benzamidine sepharose. This approach makes it possible to obtain a total fraction consisting of both active enzymes and proenzymes. The level of serine proteases in the liver of control rats was found to decrease with age. Thus, the levels of serine proteases were 0.291 ± 0.014, 0.135 ± 0.006, and 0.111 ± 0.003 mg.g of total liver weight in the liver of control rats of Groups # 1, # 2, and # 3, respectively. In contrast, the level of serine proteases in HM rats was found to be higher in the old animals. This parameter was 0.462 ± 0.023, 0.520 ± 0.026, and 0.541 ± 0.027 mg.g of total liver weight of the rats of Groups # 1, # 2, and # 3, respectively (Figure 3). Our results revealed that HM caused changes in the qualitative composition of serine proteases in the liver of rats (
Levels of matrix metalloproteinases
The levels of several MMPs (MMP-1, MMP-2, and MMP-3) were also determined. In the rats of Group # 1, we observed an increase in MMPs: the levels of MMP-1, MMP-2, and MMP-3 increased 2.35 times, 1.29 times, and 1.48 times, respectively, compared with the corresponding control values (
Discussion
Proteolysis plays an essential role in maintaining overall cellular health. Under physiological conditions, the rate of proteolytic processes is adeptly controlled to avoid pathological consequences13. In the absence of compensatory mechanisms to normalize proteolytic imbalance, intracellular pathways can be disrupted, leading in many cases to cell death due to necrosis and/or apoptosis. In the current study, we have demonstrated that HM affects the proteolytic balance in the liver. This is manifested by an increase in the total proteolytic activity, the activity of metal-dependent and serine proteases. An increase in the total proteolytic activity in the liver of HM rats may be one of the mechanisms involved in the progression of disorders associated with HM, not only in the liver but also in other tissues and organs. Accumulating evidence suggests that even moderately elevated homocysteine level is a strong independent risk factor of atherosclerosis and thrombotic events14, 15. Homocysteine can mediate its harmful effects through various mechanisms, for example, through endothelial dysfunction, increased coagulation, and impaired fibrinolysis.
Structural modifications of clotting factors induced by homocysteine or its derivative thiolactone appear to be among the most important causes of homocysteine-mediated abnormalities of hemostasis16, 17. A large number of researchers have focused their scientific attention on the effects of homocysteine on hemostatic factors that are present in the bloodstream. However, the question of whether HM affects these factors at the place of their synthesis remains open and needs to be clarified. It seems that the liver might play an essential role in the HM-induced disorders of hemostasis through the alteration of the functional activity of the coagulation factors that are generally serine proteases18. Based on all of the above, we investigated the content and quantitative composition of serine proteases in the liver of HM rats. A significant increase in the level of serine proteases with a molecular weight of less than 30 kDa and a simultaneous decrease in the level of proteins with a molecular weight of greater than 50 – 70 kDa may be the result of enhanced proteolysis and degradation of serine proteases.
Among the consequences of the degradation of serine proteases, including hemostasis factors, is the appearance of molecules that have lost their biological activities and cannot realize their inherent functions. Meanwhile, some of these truncated molecules can retain enzymatic activity because of the preservation of the active center19. However, because of the disruption of their native structure, they can avoid inhibition by canonical inhibitors. For these reasons, the formation and accumulation of degraded serine proteases in the liver of HM rats appear to be part of the set of molecular mechanisms underlying the progression of the disease and the development of serious complications. The obtained data reflect the involvement of MMPs in the development of proteolytic imbalance in the liver. Excessive or inappropriate activation of MMP creates a proteolytic environment for the degradation of cellular proteins.
In this case, MMPs are assumed to perform noncanonical functions, such as the activation of other enzymes, including serine proteases. This assumption is partially consistent with an increase in the activity of serine proteases in the liver of HM rats. The increased level of MMPs may be a consequence of oxidative stress, which is typical for the pathogenesis of HM20. According to the literature, reactive oxygen species through the regulation of redox-sensitive pathways may affect the expression of both proteases and tissue inhibitors of metalloproteinase (TIMPs), which results in abnormal MMP activity21. Moreover, homocysteine may activate the zymogen pro-MMPs to the active forms. Although MMPs are known to exert an antifibrotic effect by regulating the turnover of ECM proteins, uncontrolled long-term activation of certain MMPs, namely MMP-3, can contribute to the development of fibrosis22. Furthermore, an increased level of MMP-2 has been confirmed to be associated with the progression of metastases23, 24. Therefore, the increase in the level and activity of metal-dependent enzymes is a negative prognostic criterion in HM disease.
Conclusions
To our knowledge, this is the first study of liver proteolysis in rats with HM. The pathogenesis of HM was shown to be accompanied by a proteolytic imbalance that was manifested by an increase in the total proteolytic activity, the activity of serine proteases and especially the activity of metal-dependent enzymes. The increase in the levels of MMP-1, MMP-2, and MMP-3 indicates the involvement of these enzymes in the amplification of proteolysis mediated by metal-dependent enzymes. Despite a significant increase in the content of serine proteases, their activity did not increase significantly. This may be because of proteolytic degradation of serine proteases, which is confirmed by changes in the qualitative composition of serine proteases. The accumulation of low-molecular-weight proteins and the decrease in the part of proteins with a molecular weight of greater than 50 kDa in the fraction of serine proteases were revealed. The present results provide additional insight into the mechanisms involved in the pathogenesis of HM. Our observations indicate that impaired proteolysis may be an important determinant in developing liver disorders. The fact that only the metal-dependent and serine enzymes were investigated in this research, and no attention was paid to the aspartic, or/and cysteine proteases may be a limitation in the comprehensive assessment of overall proteolytic homeostasis in the liver. Further research is required to elucidate the molecular mechanisms underlying proteolytic imbalance in the liver in HM and the involvement of other proteolytic systems.
Abbreviations
HM: hyperhomocysteinemia
MMP: matrix metalloproteinases
Acknowledgments
This article was derived from a research project approved by the Esfarayen University of Medical Sciences, which was conducted with the financial support of this university. The researchers would like to express their gratitude to the University Research Council and all patients, who participated in this study.
Author’s contributions
RN, wrote the article, data interpretation; HY, KO, and KD, carried out the experiments; HT and GYu, manuscript review; VT and SO, experimental design; OL and MO, supervised the study. All authors read and approved the final manuscript. All authors read and approved the final manuscript.
Funding
None.
Availability of data and materials
Data and materials used and/or analyzed during the current study are available from the corresponding author on reasonable request.
Ethics approval and consent to participate
This study was conducted in accordance with the amended Declaration of Helsinki. The study was approved by the Ethical Committee of Taras Shevchenko National University of Kyiv.
Consent for publication
Not applicable.
Competing interests
The authors declare that they have no competing interests.