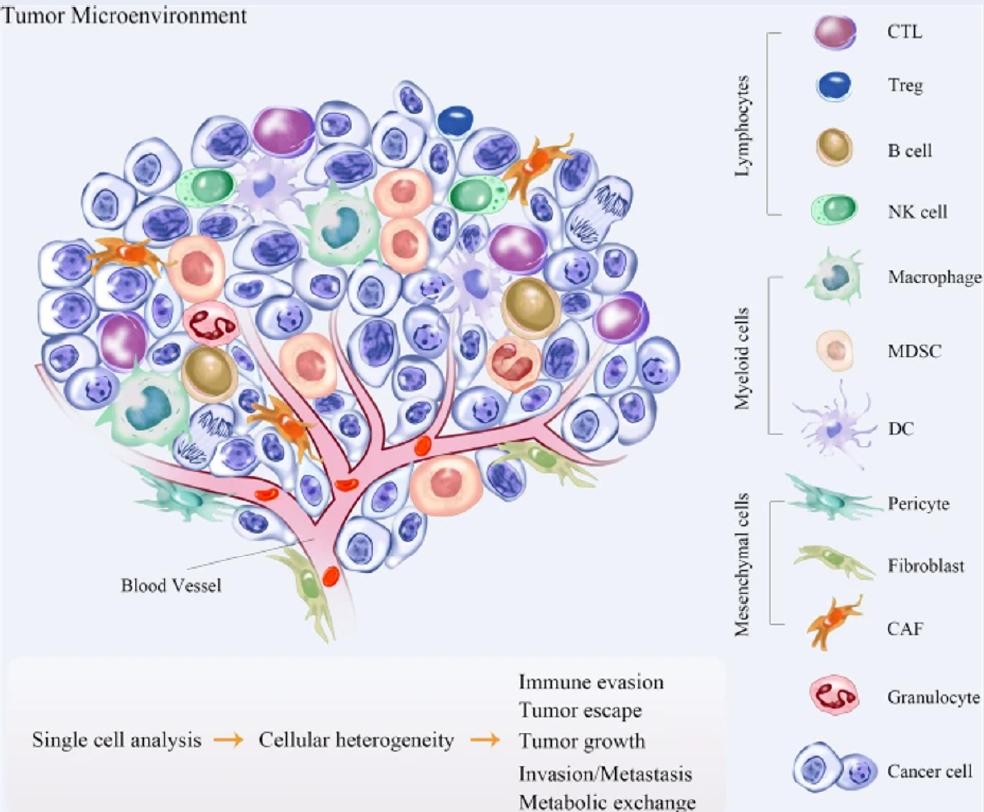
Reprogramming macrophages toward M1-like phenotypes in the breast cancer microenvironment using mesenchymal stromal/stem cells: A review
- Department of Biomedical Science, Advanced Medical and Dental Institute, Universiti Sains Malaysia, Bertam, 13200 Kepala Batas, Pulau Pinang, Malaysia
- Preparatory Centre for Science and Technology, Universiti Malaysia Sabah, Jalan UMS, 88400 Kota Kinabalu, Sabah, Malaysia
- Breast Cancer Translational Research Program (BCTRP), Advanced Medical and Dental Institute, Universiti Sains Malaysia, Bertam, 13200 Kepala Batas, Pulau Pinang, Malaysia
Abstract
Breast cancer is the most common type of cancer in women worldwide and is the type of cancer with the most frequent high mortality rate for women in 110 countries. Treatment methods offered can have both short- and long-term effects on mobility, function, and quality of life. Improvement in treatment is essential to increase the survival rate and life expectancy. Macrophages in the breast cancer tumor microenvironment (TME) are known as tumor-associated macrophages (TAMs) and are the most common leukocyte population in mammary cancer. TAMs exhibit a phenotype similar to that of M2-like macrophages and secrete a variety of chemokines, cytokines, and growth factors, such as CCL2, CCL18, IL-10, VEGF, and PDGF, which are involved in cancer progression and metastasis and trigger drug resistance during cancer therapies. Hence, high infiltration of TAMs in breast cancer patients is closely associated with a poor clinical prognosis. Mesenchymal stromal/stem cells (MSCs) have been demonstrated by various studies to modulate immunomodulatory responses and reprogramming of TAMs to M1-like macrophages. MSCs skew naïve macrophages to a proinflammatory M1-like polarized state, which can alter the TME landscape. Hence, reprogramming TAMS to an M1-like phenotype with MSCs is a good strategy to enhance commonly used immunotherapies for the improvement of clinical outcomes among cancer patients. This present review discusses the potential of targeting TAMs by reprogramming macrophages using MSCs to increase antitumor responses in breast cancer.
Introduction
Cancer is one of the primary causes of death from noncommunicable diseases (NCDs) along with cardiovascular and respiratory disorders. NCDs are becoming the world's leading cause of death and morbidity worldwide1. People of all ages are at risk of developing NCDs even before birth, even though these diseases are commonly associated with elderly people. NCDs can begin during infancy and progress throughout childhood, adolescence, and old age2. When both sexes are considered, female breast cancer exceeded lung cancer as the most diagnosed cancer worldwide in 2020, according to Sung ., with an expected 2.3 million new cases (11.7%) followed by lung cancer (11.4%)3. Female breast cancer is also the fifth leading cause of cancer mortality globally and ranks first in mortality among women in 110 countries3. Transitioned countries have 88% higher incidence rates than transitioning countries (55.9 and 29.7 per 100,000, respectively), but a 17% greater mortality rate is recorded for women in transitioning countries than women in transitioned countries (15.0 and 12.8 per 100,000, respectively)3. Diagnosis at a younger age has been linked to a poor clinical outcome, possibly due to being of a more aggressive subtype, such as triple-negative or HER2-positive breast cancer, or due to delayed discovery and presentation at an advanced stage4.
Breast cancer is not only limited to females but also occurs in men, which account for relatively 1% of all breast cancer cases, and there is still limited knowledge on the risk in men5. However, breast cancer cases among men are commonly hormone receptor-positive and may be more sensitive to hormonal therapy5. Surgery, radiation therapy (RT), chemotherapy (CT), endocrine therapy (ET), and targeted therapy are common treatments for breast cancer. Adjuvant therapy is normally introduced after surgery to ensure that the respective patients fully recover and minimize the risk of metastases6. Short- and long-term effects may occur due to the side effects of these therapies, which can affect the mobility, function, and quality of life of the patient. Local trauma from surgery or RT may cause swelling, fibrosis, nerve and muscle damage, resulting in pain, loss of shoulder range of motion and strength, and lymphedema7, 8. Peripheral neuropathy, osteoporosis, and fatigue were also reported in patients following CT7, 8. Increased survival rates and life expectancy of breast cancer patients can be achieved with early detection and increased effectiveness of current treatment approaches7, 8.
Nontumor cells in the tumor microenvironment (TME) can develop different mutations during cancer progression and may cause drug resistance during cancer therapies9, 10. The nontumor cells in the TME can also change several biological properties when they are coopted by tumor cells since there is a delicate balance between their inhibitory action and tumor-promoting factors9, 10. Furthermore, various types of cells are found in the TME, and their compositions differ based on tumor type. Tumor cells, blood vessels (endothelial cells and pericytes), lymphatic vessels (lymphedothelial cells), adipocytes, fibroblasts, lymphocytes, and various stem and progenitor cells reside in the TME11. The population of macrophages is relatively high in the breast cancer TME and is identified as tumor-associated macrophages (TAMs). In breast cancer, macrophages can make up as much as 50% of the total cell mass. The high density of TAMs promotes breast cancer metastasis and correlates with poor clinical prognosis in breast cancer patients12, 13. Intense research has been carried out in recent years to target TAMs as therapeutic strategies to prevent tumor progression and metastasis12, 13. Coculturing mesenchymal stromal/stem cells (MSCs) with naïve macrophages skewed them to an M1-like state and elicited pro-inflammatory responses14. However, manipulation of macrophage types by MSCs necessitates extensive research, especially on the polarization states of macrophages in different disease platforms14. Therefore, the current review aims to discuss the potential of reprogramming macrophages using MSCs to target TAMs in the breast cancer TME.
Tumor-associated macrophages (TAMs)
Background
The high abundance of immune cells in the tumor was initially identified by Dr. Rudolf Virchow in 1983, who suggested a link between inflammation and the origin of cancer12. These immune cells secrete various cytokines, chemokines, growth factors, and inflammatory mediators12. Macrophages are cells of innate immunity, which is the first line of defense against pathogens10. Macrophages regularly patrol and monitor their surroundings to engulf any foreign pathogens and are also involved in the antitumor response, as they can identify nonself cells and phagocytize them10, 15. Macrophages and dendritic cells (DCs) are potent antigen-presenting cells (APCs) that engulf, process, and present foreign antigens as peptides that bind with either major histocompatibility complex (MHC) class I or II on their cell surfaces16. T-cell activation is triggered when the T-cell receptor (TCR) expressed by CD4 T cells recognizes the peptide-MHC complex presented by APCs and the engagement of costimulatory molecules, either B7-1 or B7-2, with CD28, which leads to IL-2 cytokine signaling16. Upon activation, T cells undergo proliferation and acquire effector functions, which include the production of effector molecules that mediate cytotoxicity, such as perforin and granzymes16. Immunodeficiency, host tissue damage in the form of autoimmunity, malignancy, and failure to eliminate invading pathogens can all be caused by poor communication between macrophages and T cells16. TAMs commonly originate from circulating monocytes recruited to the tumor site, which then differentiate into macrophages17. Circulating monocytes are derived from hematopoietic stem cells in the bone marrow17. In addition, TAMs are also derived from embryos, such as tissue-resident macrophages in the liver (Kupffer cells), lungs (alveolar macrophages), and brain (microglia)17. This group of TAMs resides in the tumor tissue and maintains itself through self-renewal17.
Phenotype of TAMs
Macrophages that infiltrate the tumor sites normally exhibit antitumoral properties but are likely to become anti-inflammatory and protumorigenic cells once they are populated in the TME18. Macrophages can be activated into either classically activated macrophages (M1 macrophages) or alternatively activated macrophages (M2 macrophages) depending on the immune response that was induced19. The classification of TAM subtypes is essential to allow the study of how different TAM subtypes affect the regulation of gene expression and modulate the biological behaviors of cancer cells19. TAMs are exceedingly plastic and heterogeneous, and their polarization reflects a continuum. As a result, polarized TAMs are now referred to as M1-like or M2-like macrophages, rather than M1 or M2 macrophages, which are oversimplified terms20. Macrophages can be activated to the M1-like phenotype following stimulation by interferon-γ (IFN-γ), lipopolysaccharide (LPS), tumor necrosis factor-alpha (TNF-α), and granulocyte-macrophage colony-stimulating factor (GM-CSF), while M2-like macrophages are activated by macrophage colony-stimulating factor (M-CSF), IL-4, IL-10, IL-13, IL-33, and/or transforming growth factor-beta (TGF-β) (Figure 1)21. M2-like macrophages can be further induced into different subtypes of M2a, M2b, M2c, and M2d under the influence of the respective stimuli (Figure 1)22. M1-like macrophages secrete proinflammatory cytokines such as IL-1α, IL-1β, IL-6, IL-12, TNF-α, and type I interferons (IFN), enabling them to participate in immune stimulation and defense against microbial infections (Figure 1)23. M2-like macrophages produce anti-inflammatory cytokines such as IL-4, IL-6, IL-10, IL-13, and TGF-β that exhibit anti-inflammatory responses and can repair damaged tissue (Figure 1)24. The plasticity of TAMs enables them to switch between M1-like and M2-like macrophages depending on their respective microenvironment25. However, TAMs possess a phenotype similar to that of M2-like macrophages, which promotes tumor growth and metastasis26. Thus, converting TAMs into an M1-like phenotype, which has an antitumor response, may alter the TME landscape, thereby improving cancer outcomes26.
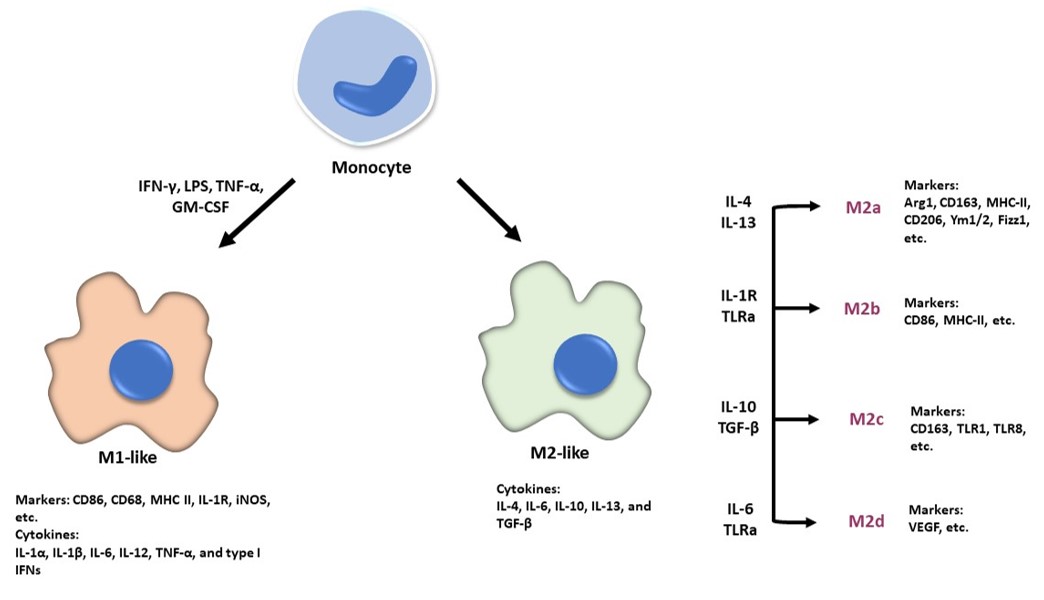
Phenotype of macrophages. Macrophages could be classified into two subtypes namely M1-like macrophages and M2-like macrophages. Meanwhile, M2-like macrophages can be sub-classified into M2a, M2b, M2c, and M2d phenotypes which are differentiated based on their secretion of respective cytokines, chemokines, and receptors. All these macrophages’ phenotypes have different functions respectively. M1-like macrophages elicit proinflammatory responses and are closely related to the Th1 response while M2-like macrophages promote tropism and tissue tolerance. Furthermore, M2a is modulating tissue repair and rehabilitation, and is associated with Th2 responses; M2b is usually involved in immunoregulation; M2c plays a critical role in phagocytosis, and M2d contributes to angiogenesis. The figure was adapted from Wang
TAMs in the breast cancer TME and therapeutic potential
Breast cancer TME
The TME can generally be divided into cellular and acellular components. In addition to tumor cells, a variety of cells, such as fibroblasts, endothelial cells, adipocytes, immune cells and neuroendocrine (NE) cells, have also been identified in the TME28. The acellular components consist of extracellular matrix (ECM), extracellular vesicles (EVs), and cytokines surrounding these cells28. Interaction between malignant and nonmalignant cells forms the TME29. Vascular endothelial cells (ECs) and pericytes, lymphatic ECs, fibroblasts, myofibroblasts, and different bone marrow-derived cells, such as macrophages, neutrophils, mast cells, myeloid-derived suppressor cells (MDSCs), and mesenchymal stromal/stem cells (MSCs), are part of the stromal cells that are nonmalignant(Figure 2)29.
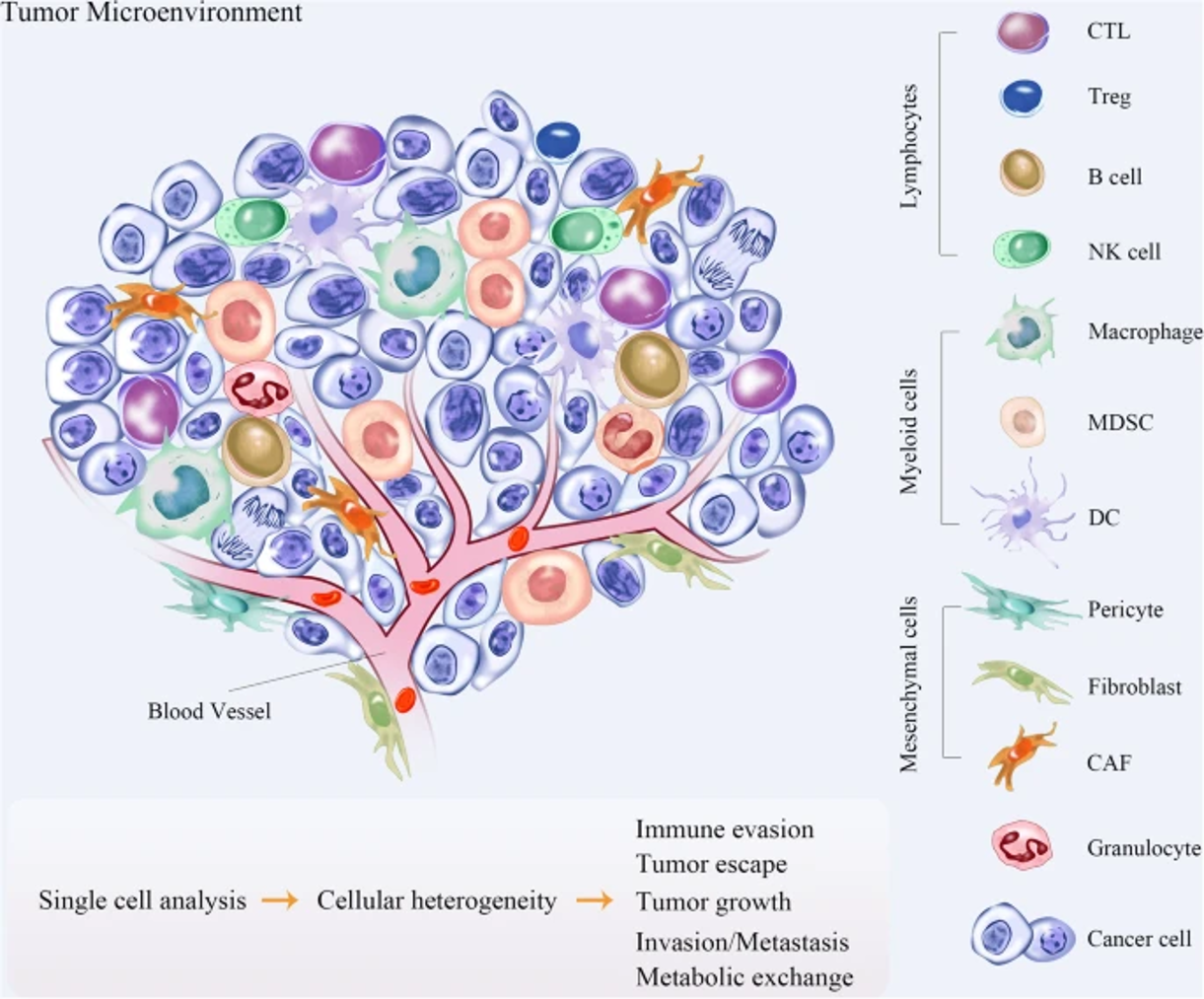
Breast cancer TME. Breast cancer TME is populated not only by cancer cells but also composed of a variety of lymphocytes, myeloid cells, and mesenchymal cells including fibroblasts, pericytes, and cancer-associated fibroblasts. The complex crosstalk between these types of cells with cancer cells in TME dictates the immune infiltration, breast cancer progression, and anti-cancer responses. The figure was obtained from Yan
Tumor cells in the TME recruit a wide array of cells that have bidirectional interactions with tumor cells31. ECs directly interact with tumor cells and are involved in angiogenesis, which contributes to tumor growth and metastasis31. Breast tumor progression is dictated by the balance between protumor and antitumor immune responses31. An increasing number of immune cells, such as CD4 T helper (Th) cells and CD8 cytotoxic T cells (CTLs), in the TME is correlated with a good prognosis in some cancers, but the presence of TAMs augments tumor growth32. Other T-cell subsets found in solid tumors, such as Th2 cells, T regulatory (Treg) cells, and Th17 cells, are involved in tumor promotion, progression, or metastasis33. The presence of other cells, such MDSCs, tumor-associated neutrophils (TANs), and cancer-associated fibroblasts (CAFs), also supports tumor growth33. The TME has started to gain popularity in recent research that focuses on cancer treatment. Understanding how the TME changes throughout tumor development could lead to the development of treatment techniques that target tumors at a certain time in their evolution34.
Fibroblasts become activated and differentiate into myofibroblasts upon tissue insult to stimulate the recovery of an injured site via the production of ECM components35. Myofibroblasts finally disappear when a wound heals, primarily due to apoptosis35. Apart from resident fibroblasts, CAFs can also be generated from other cells that are specifically mesoderm-derived, such as pericytes, adipocytes, hematopoietic cells, MSCs, epithelial cells, and ECs36. The presence of CAFs in the TME stimulates tumorigenesis through the production of growth factors that elicit angiogenesis, epithelial-mesenchymal transition (EMT), immunosuppression, tumor cell proliferation and invasion, and metastasis37. The significance of adipocytes in the breast TME arises as there is a high concentration of fatty cells compared to other organs that characterize the tissue and form tumoral mammary adipose tissue (MAT)38. Adipocytes can stimulate tumor cell growth and survival, crosstalk with the surrounding cells, and release fatty acids, which are metabolized by tumor cells and used as an energy source39. In addition, tumor-associated adipocytes (TAAs) aid in the recruitment of macrophages to the TME and their polarization into the M2-like configuration via CCL2, IL-1β, and CXCL1239. Adipose tissue can be classified into white, brown, and brite according to origin, structure, and function40. White adipocytes are the primary energy storage cells, while brown adipocytes can produce heat40. The origin and function of brite adipocytes are less clear and are currently being debated40. Brite adipocytes have the mixed characteristics of both white and brown adipose tissue40. Accumulated fatty acids released by dysfunctional adipose tissue will be taken up into the tumor and useful for energy regeneration through β-oxidation, as fatty acids are the major source of ATP in the tumor41. In addition, adipose tissue residual may also contribute to drug resistance during chemotherapy42. Activated myeloid progenitor cells differentiate into MDSCs following the secretion of proinflammatory cytokines during chronic inflammation43. These cells either elicit the recruitment of Treg cells into the TME or differentiate into TAMs43. MDSCs in the breast TME promote cancer progression by supporting EMT of tumor cells43. MSCs are part of nontumor cells recruited into the inflammatory TME44. These recruited MSCs preserve their counterpart naïve MSC differentiation capacity and stromal surface markers, but these tumor-associated MSCs (TA-MSCs) assist tumor growth44. TA-MSCs can recruit immunosuppressive cells, support EMT and tumor cell proliferation, and increase the proportion of cancer stem cells45.
The ECM is a collection of cell-secreted molecules that provides biochemical and structural support to cells, tissues, and organs37. The mechanical properties of the ECM are determined by the structural requirements37. Apart from exerting an effect on cell behavior, ECM may also contribute to tumor progression based on the composition, stiffness, topography, and microarchitecture of the ECM37. The ECM plays a role in evolution and cancer spreading because the adhesion of the cell to the ECM controls the movement in and out from the TME46.
TAMs in the TME induce breast cancer progression and resistance to treatment
Hypoxia is a primary microenvironmental stressor that is associated with tumor progression-related phenomena such as tumor cell proliferation, cell migration, neoangiogenesis, and metastatic transformation47. The rapid proliferation of transformed cells triggers hypoxia, a common feature of the TME at a very early stage of tumor development48. Hence, tumor neoangiogenesis causes rapidly dividing tumor cells to overcome hypoxia for growth and survival48. Macrophages are known to prefer the hypoxic regions in which the hypoxic microenvironment is predicted to have the ability to prime macrophages to serve protumoral functions49. TAMs immobilize between transient (avascular and nonnecrotic) and prenecrotic areas of human breast tumors, as well as prostate, endometrial, ovarian, and lung carcinomas, according to clinical data50. A greater number of M2-like TAMs have been found in human endometrial, breast, prostate, and ovarian carcinomas51. Based on an study by Tripathi ., certain soluble factors are released by hypoxia-primed breast cancer cells, which may assist in the directional migration of macrophages51. Polarization of THP-1-derived macrophages to M2-like macrophages was observed after incubation with conditioned medium from hypoxic breast cancer cells. The results from the study suggest the possibility that Oncostatin M and Eotaxin stimulate the infiltration of macrophages and M2-like polarization51.
A study by Li . revealed that endocrine-resistant tumor cells promoted M2-like macrophage polarization, while M2-like polarized TAMs enhanced endocrine resistance in tumor cells, producing a positive feedback loop between TAMs and breast cancer cells52. Macrophages cultured in conditioned media of tamoxifen-resistant cells (MCF7-R) significantly enhance the polarization of macrophages to M2-like cells compared to conditioned media from tamoxifen-sensitive cells (MCF7-S)53. By changing amino acid metabolism in the environment, endocrine-resistant breast cancer cells stimulate the mTORC1-FOXK1 pathway in macrophages, boosting M2-like polarization and CCL2 production53. Secretion of CCL2 by TAMs activates the PI3K/Akt/mTOR signaling pathway and promotes endocrine resistance52. In addition, CCL2 stimulates monocyte and macrophage aggregation in the TME (Figure 3)52. The transition from monocytes to TAMs stimulates the development of an endocrine-resistant milieu52. Resistance to tamoxifen, a common worldwide endocrine therapy, may limit the effectiveness of treatment using endocrine therapy in breast cancer53. A feedback loop between TAM-released CCL2 and activated PI3K/Akt/mTOR signaling in cancer cells could be suggested as the mechanism of resistance54. Apart from that, aggregation of monocytes into the TME by CCL2 can also promote the formation of the endocrine-resistant microenvironment52.
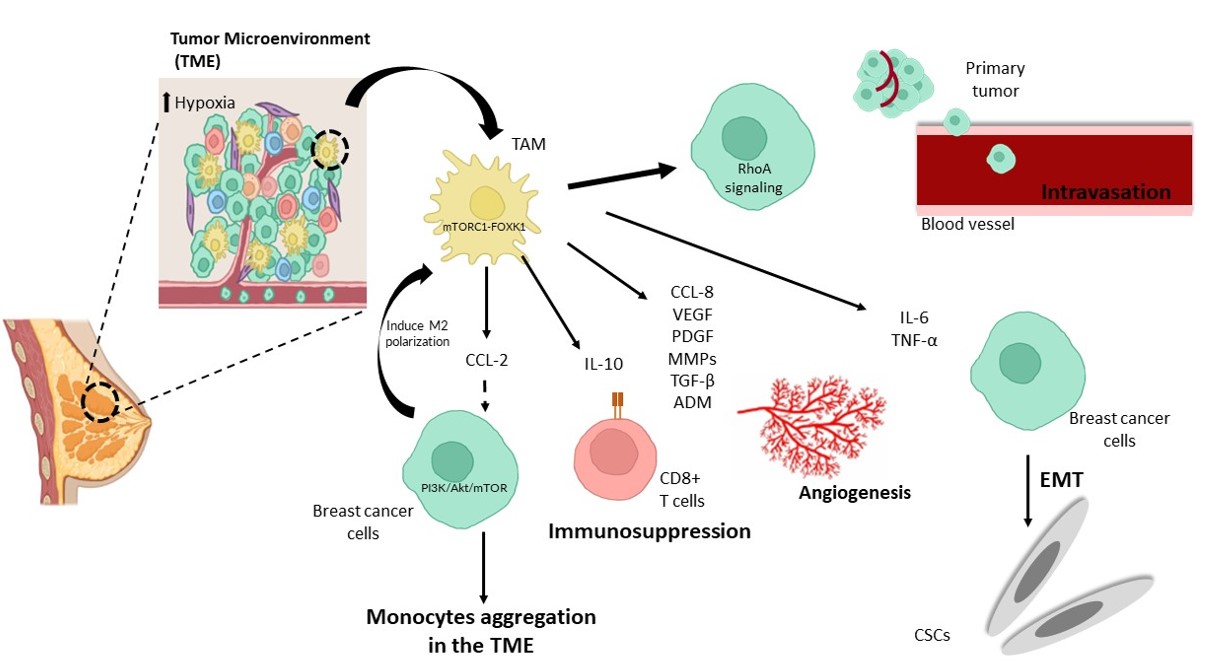
The role of TAMs in TME by inducing breast cancer progression and resistance to treatment. Cancer hallmarks are biological abilities gained during the multistep evolution of human malignancies. TAMs secrete various cytokines in breast cancer TME which can either directly or indirectly promote cancer growth and resistance in treatment via immunosuppression, angiogenesis, EMT, intravasation, and monocytes aggregation in TME.
CCL18 is another chemokine secreted by TAMs that can promote breast cancer cell metastasis55. According to a study by Lin., infiltration of TAMs secreting CCL18 is directly associated with microvascular density in human breast invasive ductal carcinoma, suggesting the possible role of CCL18 in promoting angiogenesis (Figure 3)56. VEGF is another angiogenic factor that plays a significant role in cancer metastasis57. TAMs and several other TME cell types have been shown to release VEGF57. Little showed that increased VEGF secretion from IL-4/IL-13 stimulated M2a macrophages, while both CCL18 and VEGF promoted breast cancer migration and invasion, possibly as a preangiogenic step57. VEGF has well-defined roles in promoting tumor cell migration through its stimulation57. PDGF, metalloproteinases (MMPs), TGF-β, and adrenomedullin (ADM) are other proangiogenic factors secreted by TAMs that can induce neovascularization (Figure 3)58.
In murine breast tumor models, Fang showed that TAMs are more immunosuppressive via inhibition of T-cell proliferation compared to tumor-infiltrating MDSCs (tiMDSCs)59. The study also showed that TAMs express higher IL-10, Arginase 1, CCL17, and CCL22, while tiMDSCs have higher gene expression of proinflammatory factors such as IL-12α, IL-1β, and CXCL11 and are more angiogenic59. As a result, specifically targeting TAMs rather than tiMDSCs might improve the immunosuppressive TME and boost cancer immunotherapy effectiveness59. The ratio of tiMDSCs/TAMs is lower in established tumors than during tumor initiation, which may be due to the hypoxic TME, which encourages the differentiation of tiMDSCs into TAMs59. IL-10 produced by macrophages suppresses the expression of IL-12 by DCs, which reduces CD8 T- cell infiltration and activity and increases chemotherapy resistance (Figure 3)60. In addition to drug resistance, IL-10 secreted by TAMs can also be responsible for increasing tumor survival by inhibiting NK and T-cellcell cytotoxic effects61. Yang showed elevated bcl-2 gene expression and STAT3 signaling in breast tumor cells62. This suggests the possibility of TAMs promoting drug resistance via the IL-10/STAT3/bcl-2 signaling pathway62.
EMT refers to the change in tumor cells from epithelial to mesenchymal features, which increases tumor cell motility and invasive capacities63. TAMs also play a role in this process by secreting inflammatory factors such as TNF-α and IL-6 (Figure 3)63. These inflammatory factors stimulate EMT by activating the TGF-β or NF-κB pathways63. TNF-α also enhances EMT by activating ROS63. IL-6 accomplishes EMT by activating the JAK/STAT3 pathway63.
Another study by Sousa demonstrated that breast cancer cell-secreted factors modulated macrophage differentiation into the M2-like phenotype64. MDA-MB-231, which produces a large amount of M-CSF, skews macrophages toward a more immunosuppressive subtype of M2c64. Additionally, increased CD200R signaling was observed in M2a macrophages differentiated in the presence of IL-4 and MDA-MB-231 conditioned medium64. Cancer cells are among cells that express CD200 other than MSCs, thymocytes, activated T cells, B cells, and DCs64. The CD200-CD200R interaction can stimulate an immunosuppressive signal by reducing inflammatory cytokines secreted by macrophages and indirectly increasing Treg and reducing effector T-cell numbers, which in turn promote tumor progression64.
A study by Qin reported a possible relationship between stress and epinephrine (E), a stress hormone with the progression of breast cancer growth via the transformation of macrophages from M1 to M265. Apart from accelerating tumor growth, the elevated presence of E in the TME can also promote the polarization of macrophages into the M2 phenotype, which in turn can support tumor progression65. The influence of the TME on the transformation of macrophages to M2 macrophages might be mediated by the expression of the ADRβ2 receptor, as the expression of the ADRβ2 receptor is positively correlated with the expression of M2 macrophages (CD206) in human breast cancer tissues65.
Although the involvement of macrophages in tumor cell entry into the vasculature has been studied and , the mechanism by which macrophages particularly alter tumor cell intravasation is still unclear66. In a study using patient-derived breast tumor cells, Roh-Johnson . discovered that macrophages could increase tumor cell intravasation by activating the RhoA signaling pathway, leading to the development of invadopodia66. Tumor cells can then intravasate by penetrating the basement membrane of the vasculature (Figure 3)66.
The therapeutic value of macrophages in breast cancer
A high density of TAMs in breast cancer patients is associated with a poor survival rate, while increased TAM infiltration is linked to a negative hormone receptor status and a malignant phenotype67. Hence, TAM invasion may be a new predictive factor in patients with breast cancer67. A prognostic biomarker is a clinical or biological trait that predicts a patient's health outcome, such as disease recurrence, regardless of treatment68. A high level of TAM infiltration in invasive breast cancer (IBC) is linked to poor clinical and pathological prognostic factors, such as high histological grade, large tumor size, ER negativity, PR negativity, and a high Ki-67 proliferating index69. Additionally, it is crucial to investigate the location of TAMs as a prognostic marker. In the tumor stroma, a higher number of macrophages (CD11c or CD163) was linked to a larger tumor size, while CD163 macrophages were found in the tumor nest and became an independent predictive marker for shorter overall survival (OS) and disease-free survival (DFS)69. In breast cancer, high TAM infiltration is linked to EMT and can be used as a negative prognostic indicator for patients with triple-negative breast cancer (TNBC)70. TNBC patients with CD163 TAM infiltration and low E-cadherin levels had a higher risk of aggressive characteristics, such as recurrence, histologic differentiation, and lymph node metastasis70. A prior study showed that patients with low CD163TAM density had considerably higher progression-free survival (PFS) and overall survival (OS) than patients with high CD163 TAM density71. In addition to CD163, TAM-related markers such as CD68 and MMP-9 can also be used as breast cancer biomarkers, but macrophage activity markers are linked to survival differently in ERand ERcancers72. MMP-9 and CD163 have long been associated with the M2 phenotype; however, Pelekanou discovered that this association does not always translate into a worse outcome in breast cancer72. In ER cancers, high CD68 expression was associated with poor survival, whereas high CD163 expression was associated with improved OS73. On the other hand, high MMP-9 expression in CD68/CD163 TAMs was linked to worse OS in ER tumors but not in ERcancers73. Markers associated with M2-like TAMs that support tumor invasion and progression are potentially useful as prognostic markers for breast cancer patients.
Current gene assays employ proliferation-related genes that are restricted to tumor cells to stratify patients into different risk groups but not prognosis-related immune cell marker genes73. Li developed a prognostic macrophage marker gene signature (MMGS) that utilizes macrophage-related genes such as SERPINA1, CD74, STX11, ADAM9, CD24, NFKBIA, and PGK173. The MMGS had a higher ability to discriminate between high-risk and low-risk groups in the hormone receptor-positive and HER2-negative subgroups73. However, further study is required to investigate the interaction between macrophage marker genes and tumor-specific genes73.
Immunotherapy has emerged as the most promising cancer treatment, with a variety of cancer immunotherapy strategies established to date, such as adoptive cellular immunotherapy, tumor vaccines, antibodies, immune checkpoint inhibitors, and small-molecule inhibitors74. The emergence of immunotherapy is due to the limitations and scarcity of standard cancer treatments 74. Unfortunately, multiple immunosuppressive signals inside the TME limit the efficiency of the current immunotherapy techniques75. As a result, novel ways to effectively remodel the different immunosuppressive cells in the TME and elicit additional antitumor immunity are urgently required 75. Targeted treatments that act on macrophages may provide innovative therapeutic techniques, in addition to acting as biomarkers76. Recent strategies to target macrophages are focusing on macrophage depletion, inhibition of monocyte recruitment in tumor tissues, and reprogramming of TAMs toward an antitumor phenotype58.
Colony-stimulating factor 1 receptor (CSF-1R) targeting is one of the strategies investigated to deplete and/or reprogram TAMs by inhibiting monocyte recruitment in tumors77. Depending on the organ-specific properties of these cells, the impact of the technique as a therapeutic approach for cancer patients may vary substantially, as discussed by Cannarile and colleagues 77. PLX3397 (pexidartinib) is the first oral and clinically tested nonspecific CSF-1R kinase inhibitor. PLX3397 is safe and effective in reducing TAMs in a variety of solid tumors78, 79. M-CSF and IL-34 are ligands of CSF-1R80. M-CSF can recruit monocytes from the circulation and differentiate them into macrophages in the context of cancer81. Once recruited to the tumor, factors in the TME promote their differentiation into TAMs 81. Inhibiting M-CSFR or CSF-1R with PLX3397 reduced the number of TAMs, circulating nonclassical monocytes, neoangiogenesis, and ascites but did not enhance survival, as observed in mesothelioma mouse models82. A concomitant drop in TAMs and an increase in CD8 T-cell numbers and functionality were observed when paired with an effective DC-mediated antitumor T-cell response via DC vaccination, which produced long-lasting tumor responses and functional antitumor immunity 82. The therapeutic efficacy of CSF-1R inhibition as monotherapy was limited in mesothelioma therefore, further studies should be carried out to evaluate the efficacy of a similar strategy in other types of cancers 82. Emactuzumab, a monoclonal antibody against CSF-1R, can selectively deplete immunosuppressive M2-like macrophages, as observed in patients with advanced solid tumors, but is inefficient in reprogramming the remaining macrophages into immunostimulatory M1-like TAMs83. Depletion of TAMs alone may not be sufficient to improve the efficacy of breast cancer treatment but requires combination with cancer immunotherapies and/or immunostimulatory agents to induce an immune response against the tumor8283.
Increasing the M1/M2 ratio is likely to slow tumor growth, making it an attractive therapeutic target technique in cancer research. Salinomycin (SAL) treatment of the murine breast cancer cell line 4T1 effectively repolarizes TAMs toward the M1 phenotype and regresses tumor growth and metastasis84. SAL is a veterinary antibiotic used to treat coccidiosis in chickens and was previously found to selectively kill cancer stem cells (CSCs)84. Another strategy that demonstrated repolarization of M2 macrophages toward M1 is by treating 4T1 cells with miR-130-containing exosomes85. A study by Yin . demonstrated TAM polarization to M1-like macrophages in the human breast cancer cell line MCF-786. Based on that study, anemoside A3 (A3), a naturally occurring (PS) compound, was shown to induce M0 macrophages and TAMs toward an M1-like immunogenic phenotype via a TLR4-dependent signaling pathway86. Hence, targeting reprogramming TAMs toward an antitumor phenotype may be useful in preventing tumor invasion and metastasis in breast cancer.
Reprogramming macrophages with MSCs
Reprogramming of macrophages
The effectiveness of immune defense relies on the degree of macrophage plasticity, in which how fast and efficient these cells alter their functional phenotype in reaction to the microenvironment and pathogenic microbes. Based on the present concept of macrophages, proinflammatory factors such as LPS and IFN-γ can program the M1-like phenotype to increase the production of proinflammatory cytokines that activate the Th1 response, facilitate complement-mediated phagocytosis, and cause type I inflammation87, 88. A similar effect can be observed on the M2-like phenotype by stimulation of anti-inflammatory cytokines, which in turn will promote anti-parasitic immune responses and wound healing processes89. Reprogramming, also known as polarization or alternate phenotype, is the process of changing the macrophage phenotype in response to the microenvironment or an active pathogen87. However, polarization and alternate phenotypes are phrases that imply a choice between two states and do not accurately reflect the true nature of reprogramming 87. Reprogramming is better suited to explain the formation of any cell phenotype since it would be more appropriate to consider that the M1-like phenotype has more M1 markers than M2 markers and vice versa 87. Normally, macrophages can be reprogrammed either to the M1-like or M2-like phenotype based on the type of pathogen detected to provide a rapid and appropriate immune response90.
Therefore, the concept of investigating macrophage plasticity by reprogramming the TAM phenotype into an M1-like phenotype is emerging as a plausible therapeutic method for cancer treatment. Research by Wanderley demonstrated that the M2 phenotype of TAMs can be reprogrammed by paclitaxel into the M1 profile via TLR4 signaling, as observed in murine bone marrow-derived macrophages (BMDMs)91. Thus, there is a potential need to explore the reprogramming of macrophages as a strategy to enhance commonly used immunotherapies to improve clinical outcomes among cancer patients91. Apart from being targeted for the treatment of disease, macrophage reprogramming is also now used as a primary determinant of the progression and/or relapse of diseases92. According to Su ., high mobility group box 1 (HMGB1), an inflammatory factor, induces the development of experimental autoimmune myocarditis (EAM) by reprogramming infiltrating macrophages toward the M1-like phenotype via TLR4-PI3Kγ-Erk1/2 pathways92.
Immunomodulation by MSCs
Mesenchymal stromal/stem cells (MSCs) can modulate both innate and adaptive immune responses93. MSCs adhere to tissue culture plastic and have a fibroblast-like morphology94. These cells are multipotent cells that enable them to differentiate into a few mesoderm-type lineages, including myogenic, adipogenic, osteogenic, and chondrogenic lineages94. MSCs were initially isolated from bone marrow and later discovered to be able to be isolated from different sources, such as adipose tissue, synovial fluid, umbilical cord blood, Wharton’s jelly, placenta, and amniotic fluid95. MSCs are also able to express specific markers, such as CD105, CD73, and CD90, with a lack of expression for CD45, CD34, and CD1493.
Immunomodulatory changes following administration of MSCs have been widely explored, but there is still limited clarification of the cellular and molecular mechanisms96. However, comparison of study results is challenging, as different features and are displayed by MSCs depending on the tissue they originated and different protocols used for administration of MSCs96. Adipose tissue and umbilical cord-derived MSCs are commonly used due to their accessibility 96. Nonetheless, certain patterns and pathways are consistent and have been demonstrated several times in which immunomodulation by MSCs is mediated by a combination of cell contact-dependent pathways and soluble factors96.
Findings from Bartholomew , who is a pioneer in demonstrating the relationship between MSCs and the immune response, showed that baboon MSCs (bMSCs) can modify lymphocyte reactivity into allogeneic cells 97. In addition, administration of MSCs resulted in increased skin graft survival97. Following this discovery, there has been intense research focusing on the immune response between MSCs and various immune cell subsets. MSCs suppress NK, B cell, CTL, Th1, Th2, and Th17 cells but support the enhancement of Treg cell number and activity98, 99, 100.
Numerous studies have investigated the therapeutic potential of MSCs for the treatment of numerous diseases, including diabetes and diseases of the bone and cartilage101, 102, 103, 104. Perinatal MSCs become a primary source of exogenous MSCs to treat cancer due to their ability to home to tumor locations, their antiproliferative impact on multiple tumor cell lines and their ability to shape the inflammatory niche via their intrinsic immune regulatory or stimulatory abilities105. Amniotic membrane (hAMSC), umbilical cord/jelly Wharton's (UC-MSC/WJ-MSC), chorionic villi (CV-MSC), and maternal decidua (DMSC) are used to isolate perinatal MSCs 105.
Dual role of MSCs in breast cancer
MSCs have the potential to be used for cell therapy and tissue engineering, as they are easily extracted and have unique immunophenotypic and immunoregulatory capacities to be used in an allogeneic setting106. Furthermore, MSCs can migrate in a precise direction to certain tissues or home, making them ideal vehicles for targeted tumor therapy106. However, the antitumor benefits of MSCs remain debatable107. This is because MSCs do have anticancer capabilities, prompting efforts to modify them for anticancer therapy, but they have also been linked to several pathways that contribute to tumor formation108. Understanding the dual role of MSCs in tumor cell proliferation is crucial since they have the potential to be an ideal target for cell treatment in a variety of cancers108. Some studies have revealed inhibitory effects of MSCs, whereas others have indicated protumor activity of MSCs, regardless of the type of cancer that raised concerns about their clinical application in oncology, as discussed by Hmadcha .109.
The homing of MSCs from bone marrow and other sources to tumor sites is induced by the tumors and secretion of several growth factors, cytokines, and chemokines in the TME110. Growth factors (PDGF, SCF, HGF, IGF-1E, and GF), angiogenic factors (βFGF, HIF1α, and VEGF), chemokines (CCL5, CCL2, CXCL12, and CCL22), cytokines and inflammatory factors (TGFβ, TNFα, IL-8, and IL-1β) are among the components that are involved in the mechanisms of MSC homing to tumor sites110.
Recruited MSCs in the TME are “educated” to develop and differentiate into tumor-associated MSCs (TA-MSCs) and CAFs111. MSCs can prevent tumor proliferation and facilitate tumor cell apoptosis108. However, there are also studies showing that MSCs can contribute to EMT stimulation, have immunosuppressive properties, support tumor vasculature, and increase the proportion of stem cells in tumors 108. Tumor start, promotion, progression, and metastasis have all been linked to TA-MSCs when exposed to tumor-mediated "education"44. TA-MSCs, in general, exhibit differentiation potential and stromal surface markers similar to those of naïve MSCs, but they assist tumor growth through similar mechanisms 44. CAFs in breast cancer are involved in angiogenesis/lymphangiogenesis, ECM remodeling, cancer-associated inflammation, and metabolic reprogramming, all of which can signal premalignancy112.
Increased proliferation and metabolic activity of breast cancer cells were demonstrated following coculture of human breast cancer cells with adipose-derived MSCs, partly due to MSC-derived microvesicles that are shed in the TME113. For the first time, Maffey . demonstrated that MSCs had a trophic influence on breast cancer cell proliferation through ionotropic purinergic signaling 113. In addition, MSCs release cytokines and chemokines in the TME that support tumor growth, such as CXCL12, which enhances tumor cell proliferation and survival, and VEGF, which promotes neovascularization in the TME114. MSCs may provide immune protection to breast cancer cells (BCCs) through their immunosuppressive capacity following the production of MSC-secreted TGF-β115. As a result, Treg cell proliferation increased, while the functions of cytotoxic CD8 lymphocytes and CD56 NK cells were suppressed115. When human MSCs were cocultured with MDA-MB-231 cells, TGF-β produced by MSCs was shown to induce directional migration and invasion of metastatic BCCs116. The subsequent release of various trophic factors, cytokines, and chemokines by MSCs contributes to the increased proliferation of BCCs, including an increased number of breast cancer stem cells (BCSCs)117. For the preservation and progressive proliferation of BCSCs, the formation of a cancer stem cell niche (CSCN) with a timely available concentration of trophic nutrients, principally given by suitably primed MSC populations, may be necessary 117. Liu showed that bone marrow-derived MSCs (BM-MSCs) accelerated breast cancer cell growth by producing cytokines that modulate BCSC populations118. Cancer cells release IL-6, which facilitates homing of MSCs to tumor sites and induces them to produce CXCL7 118. CXCL7 produced by MSCs interacts with cancer cells via the CXCR2 receptor, causing the release of multiple cytokines, such as IL-6 and IL-8. IL-8 binds to the CXCR1 receptor on CSCs, prompting them to proliferate and invade 118. BCSCs and colon CSCs have been shown to be regulated by IL-6 118. In addition to regulating CSCs, IL-6 produced by cancer cells interacts with MSCs, causing them to secrete more CXCL7 that generates a positive feedback loop 118. Cancer stem cells (CSCs) are a tumor subpopulation that is responsible for tumor metastasis and chemo- and radiotherapy resistance, potentially contributing to tumor relapse107. CSCs have long been thought to be an original source of cancers and are responsible for homing MSCs to primary tumor growth sites by secreting IL-6, which can suppress other responses in the tumor niche119.
Exosomes produced by TA-MSCs in humans and mice facilitated rapid breast cancer development as monocytic myeloid-derived suppressor cells at tumor sites differentiate into highly immunosuppressive M2-polarised macrophages120. MSCs supported breast cancer growth via their immunosuppressive effects121. BM-MSCs have been reported to inhibit the proliferation and migration of PBMCs toward BCCs and suppress NK and CTL functions. Hence, MSCs can provide immune protection to BCCs 121.
In contrast, Leng showed inhibition of breast cancer growth in both administered human umbilical cord-derived MSCs (hUC-MSCs) and in coculture of hUC-MSCs with MDA-MB-231 cells122. Additionally, culture medium from hUC-MSCs has been proven to reduce MDA-MB-231 cell proliferation, migration, and angiogenesis and induce DNA damage by blocking the Stat3 pathway, as shown by bioluminescence imaging technology123. The Stat3 signaling system plays a role in tumor growth and breast cancer-derived stem cell survival123. The most widely recognized mechanism by which MSCs exhibit therapeutic benefits is their interaction with the tumor cell cycle109. MSCs can trigger cell cycle arrest and halt cancer progression via inhibition of proliferation-related signaling pathways such as phosphatidylinositol 3-kinase/protein kinase B (PI3K/AKT)109.
The original source of MSCs should be considered when determining the antitumor effect of MSCs, as hUC-MSCs have previously been shown to inhibit glioblastoma proliferation but not adipose tissue-derived MSCs124. Various studies that have been conducted thus far indicated that similar levels of efficacy and desired outcomes have not always been achieved125. Apart from MSC tissue sources, MCSs' ability to promote or repress tumor formation may be influenced by experimental tumor mouse models, MSC dose or timing of MSC therapy, cell delivery mechanism, control group selection, and other experimental variables109. In addition, autologous MSCs can be affected by a patient's disease status, which limits their use125. Hence, it is crucial to gather information about the characteristics of MSCs obtained from various sources, as well as the influence of the host (patient) medical conditions on MSCs when involving clinical trials, to ensure the safety and efficacy of cell-based therapies using MSCs 125. Standardizing the dose and treatment timing of MSCs and hematologic malignant cells when they are cocultured for studies involving or injected into cancer mouse models is also crucial to safely and successfully utilizing MSCs for further clinical applications108.
Polarization of macrophages with MSCs
The plasticity of macrophages enables their programming via exposure to certain stimulation factors. Apart from that, the capacity of MSCs to modulate the immune system has been widely demonstrated via their immunomodulatory effect on monocytes/macrophages, T cells, B cells, NK cells, and dendritic cells96. The capability of MSCs to regulate the function of macrophages has become clear in recent years126.
The immunomodulatory ability of MSCs is not only regulated by direct cell-to-cell contact but can also occur through paracrine action by soluble factors127. MSCs are used to convert macrophages into the anti-inflammatory M2 phenotype and induce a suppressive immune response103. Kim and Hematti cultured macrophages derived from human peripheral blood monocytes for seven days without any addition of cytokines to generate macrophages and then cocultured them with BM-MSCs for another three days128. Increased expression levels of CD206, IL-6, and IL-10 but lower levels of IL-12 and TNF-α were observed, indicating a macrophage shift toward alternatively activated macrophages or M2 macrophages following exposure to MSCs 128. Shin . demonstrated that macrophage regulation mediated by human umbilical cord blood-derived MSCs (hUCB-MSCs) offers therapeutic potential in rheumatoid arthritis (RA) patients101. hUCB-MSCs polarized macrophages toward an M2-like phenotype via TNF-α-mediated activation of cyclooxygenase-2 (COX-2) and TNF-stimulated gene-6 (TSG-6)101. Similarly, adipose-derived (AD)-MSCs increased M2 macrophage polarization via the COX-2-PGE2 pathway, which protected against heart injury in diabetic cardiomyopathy (DCM) rats129. He . also showed that MSCs can induce M2 polarization of macrophages both and with the aid of exosomes derived from MSCs130. A time-dependent increase in the number of both RELM-α, an M2 macrophage marker, and CD68, a surface marker for macrophages, was observed following systemic injection of bone marrow MSCs (BMMSCs) and jawbone marrow MSCs (JMMSCs) into mice130.
A challenge involving research is that systemically injected MSCs are confined in the lungs and have a short lifespan; only less than 0.1% of the infused cells are detected in other organs131. Coculture of BMMSCs and JMMSCs showed a higher number of both CD14CD163 and CD206 macrophages and increased levels of IL-10 but lower levels of TNF-α by the macrophages130. The skewing of macrophages to the M2 phenotype induced by MSCs may be beneficial to help in reducing arthritic inflammatory responses and promoting wound healing and may potentially be used for the optimization of bone fracture therapies101, 104, 130. However, enhancement of the M2-like phenotype in the TME promotes tumor progression.
More recent studies have shed light on the tendency of MSCs to promote macrophage polarization to the M2-like phenotype when they are initially in the M1 activation state102, 104. According to a study on type 2 diabetes mellitus (T2D) by Xie ., UC-MSCs can impair insulin resistance through the production of IL-6, which promotes M2 polarization in response to LPS-stimulated macrophages (M1 macrophages) 102. Lu . found that exposure of primary mouse MSCs to polarized M1 macrophages shifts macrophages from M1 to M2 early in osteogenesis with a better pro-osteogenic capacity104. Additionally, MSCs that are in the initial environment of M1 signaling are more potent in producing higher levels of PGE2 and other anti-inflammatory signals that are involved in the modulation of macrophage transition from the M1 to M2 phenotype104.
Vasandan . carried out a study to investigate MSC-macrophage crosstalk at each level of macrophage activation, as they postulated that the precise functional state of macrophages at the MSC interface can considerably influence therapeutic results following MSC transplantation14. From the study, human bone marrow MSCs skew THP-1-stimulated naïve macrophages to an M1 activation state, reducing already activated M1-like macrophages by shifting them to M2-like activation and enhancing M2 activation in readily activated M2-like macrophages (Figure 4)14. MSCs did not polarize naïve macrophages to the M2-like state, which is the predominant subtype in tumors, following MSC cocultures14. Hence, a study focusing on the interaction at a distinct stage between MSCs and monocytes/macrophages is crucial, as macrophages come in a variety of functional modules in a variety of disease contexts.
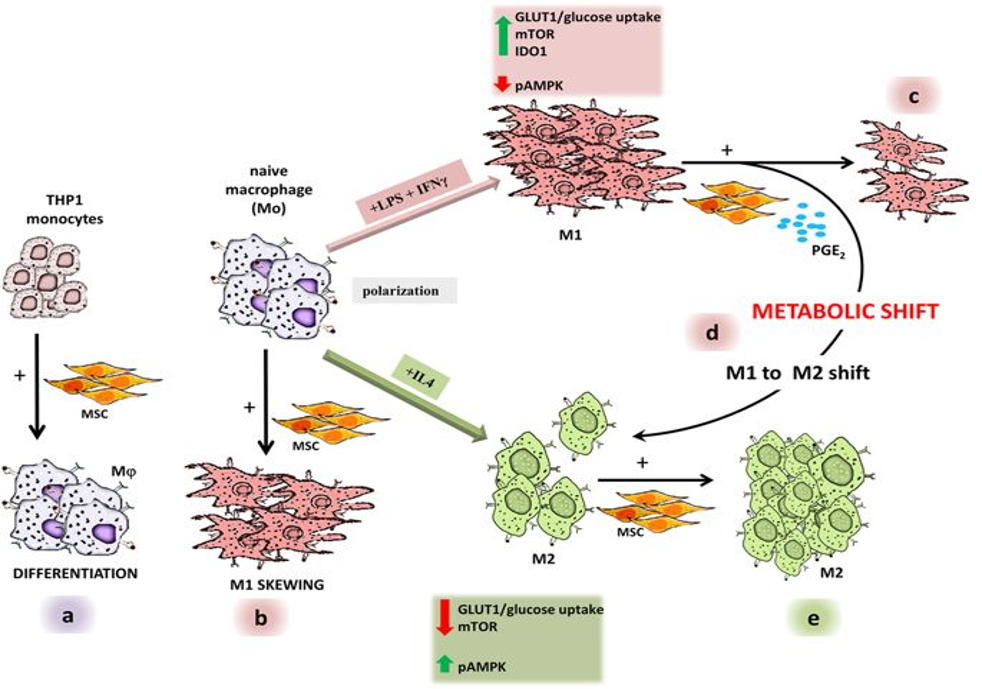
MSCs modulate macrophages phenotypes differentiation. MSCs regulate (a) conversion of monocyte to macrophage, (b) stimulate naïve macrophages to M1-like macrophages and (e) elicit the activation of M2-like macrophages. Furthermore, MSCs have the capability (c) to reduce M1-like macrophages, (d) deviate them to become M2-like macrophages via PGE2 dependent manner. The illustration was obtained from Vasandan
Other than cell‒cell interactions, conditioned medium from MSCs can also mimic the actions of entire cells via paracrine-secreted components from the MSCs, which are referred to as the secretome132. The secretome can include cytokines, chemokines, growth factors, anti-inflammatory factors and even proteins conveyed by MSC-derived extracellular vesicles (MSC-EVs)133. Exosomes (vesicles with a diameter of 50 – 150 nm), microvesicles (vesicles with a diameter of 100 – 1000 nm), and apoptotic bodies (vesicles with a diameter of 50 – 4000 nm) are the three classic categories of EVs based on their sizes and biogenesis methods132. Exosomes are created by the intracellular body pathway, in which they are released into the extracellular environment via exocytosis by disintegrating multivesicular bodies (MVBs) after binding to the lysosome or cell membrane. Late endosomes differentiate into MVBs, which contain intracellular vesicles. Early endosomes develop when the cell membrane is invaginated, and they connect with vesicles created by budding of the Golgi apparatus to form late endosomes134. Exosomes can carry proteins, lipids, and miRNAs, which, among other things, help to regulate cellular activity and facilitate intercellular communication134. Tetraspanins (CD9, CD81, CD63), HSP60, HSP70, TSG101, and Alix are protein markers found on the surface of exosomes134.
MSC-EVs isolated from the secretome can reproduce the effects of MSCs and regulate macrophage polarization132, 135. Lo Sicco . showed that macrophages efficiently internalized MSC-EVs, causing them to change from an M1 to an M2 phenotype135. Similarly, Zhao found that MSC-derived exosomes (MSC-Exos) altered the polarization of M1 macrophages to M2 macrophages, and the study also demonstrated that miR-182 in MSC-derived exosomes targets the TLR4/NF-κB/PI3K/Akt signaling cascades to influence macrophage polarization136. Injection of MSC-Exos promoted increased mRNA expression of M2 macrophage markers but inhibited the mRNA expression of M1 macrophage markers in the myocardial tissue of model mice based on a study by Shen and He137. From the study, MSC-Exo successfully transformed the polarization state of LPS-induced RAW264.7 macrophages from the M1 phenotype to the M2 phenotype and miR-21-5p is believed to regulate the polarization of macrophages to the M2 phenotype137. miRNAs have important functions in immune regulation and can have dual activities on inflammation138. However, studies have mainly focused on the role of MSC-Exos in altering the polarization of M1 macrophages to M2 macrophages but have still limited information on their effect on naïve macrophages.
The ability of MSCs to control macrophage polarization through direct cell‒cell interactions or paracrine effects makes additional study of macrophage polarization and phenotype after exposure to MSCs very interesting132. MSCs could potentially be used in cancer therapies to reprogram macrophages toward M1 antitumor phenotypes104. However, macrophage reprogramming must be focused on specific macrophage polarization states to avoid further increases in protumor M2-like TAMs when carrying out future studies to explore macrophage reprogramming with MSCs. Additionally, future research into the response of innate immune cells to MSCs may provide new insight into the exact events that occur following MSC delivery, perhaps boosting the efficacy of MSCs administered intravenously in clinical trials139.
CONCLUSIONS
Targeting TAMs, which are a part of the TME, is indeed a promising method in the treatment of breast cancer, as TAMs can not only promote tumor growth and metastasis but also trigger cancer resistance. Improvements in treatment are needed to enhance survival rates and life expectancy for breast cancer patients. Additionally, there is a high population of TAMs in mammary tumors compared to other populations of protumor cells, and these TAMs exhibit a more M2-like phenotype. Hence, M2-like TAMs in the TME are useful as prognostic markers and therapeutic targets for breast cancer treatment. Reprogramming TAMs toward the M1-like phenotype may increase antitumor activity against breast cancer cells and can be used as a strategy to enhance commonly used immunotherapies to improve clinical outcomes among breast cancer patients. MSCs have an immunomodulatory effect not only on T- and B-lymphocytes, NK cells, and DCs but also on macrophages. Cell‒cell interactions and paracrine action via soluble factor secretion are two ways in which MSCs modulate the immune response. MSCs can skew naïve macrophages to M1-like macrophages. Hence, reprogramming TAMs into the M1-like phenotype by exposing naïve macrophages to MSCs should be further explored to increase antitumor responses in the breast cancer TME. However, improvements are still needed in future studies. research involving the characterization of macrophage polarization and phenotype during coculture with MSCs or when exposed to their secretome only is required to prevent further stimulation of M2-like TAMs that support tumor progression instead. Future research into the response of innate immune cells to MSCs could provide new insight into the precise events that occur after MSC delivery, perhaps boosting the efficacy of MSCs supplied intravenously in clinical trials.
Abbreviations
APCs: Antigen presenting cells, BM-MSCs: Bone marrow-derived MSCs, CSCs: Cancer stem cells, CT: Chemotherapy, CTLs: Cytotoxic T cells, DCs: Dendritic cells, ECM: Extracellular matrix, EMT: Epithelial-mesenchymal transition, EVs: Extracellular vesicles, hUCB-MSCs: human umbilical cord blood-derived MSCs, IFN-: interferon-, LPS: Lipopolysaccharide, MDSCs: Myeloid-derived suppressor cells, MHC: Major histocompatibility complex, MSCs: Mesenchymal stromal/stem cells, MSC-Exos: MSC-derived exosome, NCDs: Noncommunicable diseases, NK: Natural killer, TAMs: Tumor-associated macrophages, TCR: T cell receptor, TME: Tumor microenvironment, TNF-: Tumor necrosis factor-
Acknowledgments
We would like to acknowledge Research University Individual grant, Universiti Sains Malaysia (1001/CIPPT/8012328) that fund this research.
Author’s contributions
NRJ, MAY, BHY and RM conceived the idea, NRJ performed the literature search and wrote the manuscript. MAY, BHY and RM gave additional inputs and rearrange the final version of the article. All authors read and approved the final manuscript.
Funding
Research University Individual grant, Universiti Sains Malaysia (1001/CIPPT/8012328)
Availability of data and materials
Not applicable.
Ethics approval and consent to participate
Not applicable.
Consent for publication
Not applicable.
Competing interests
The authors declare that they have no competing interests.