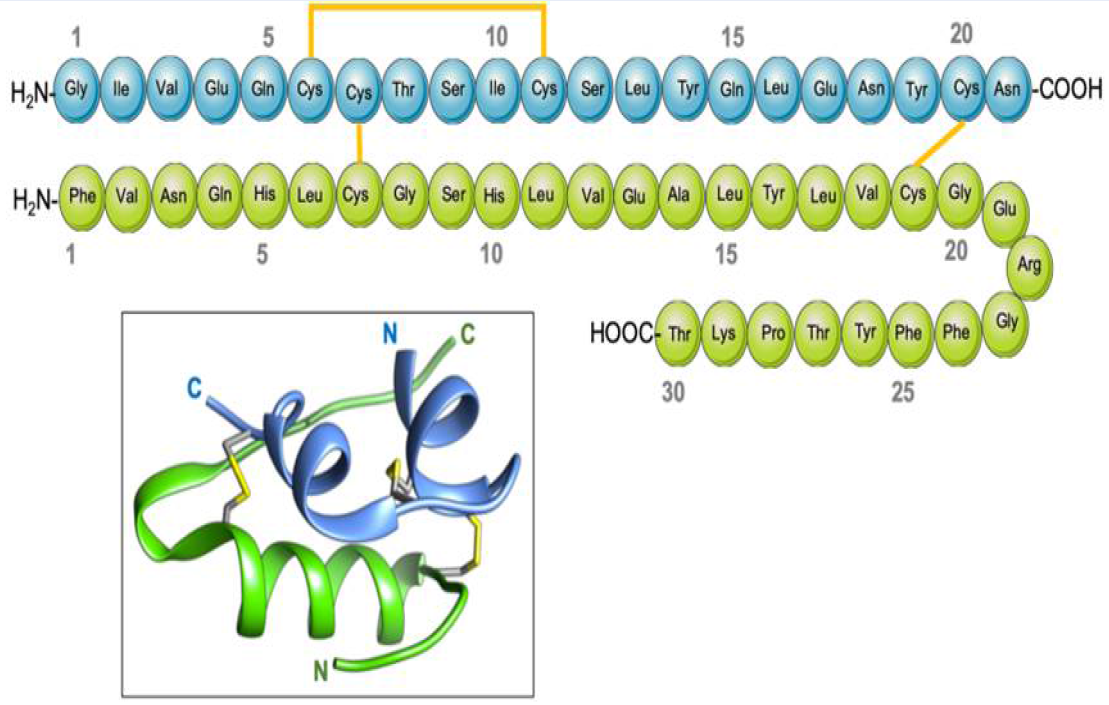
Human Insulin: History, Recent Advances, and Expression Systems for Mass Production
- Department of Biotechnology, Kinnaird College for Women University, Lahore, Pakistan
- Department of Zoology, PMAS, Arid Agriculture University, Rawalpindi, Pakistan
- Department of Botany, PMAS, Arid Agriculture University, Rawalpindi, Pakistan
- Department of Biotechnology, Faculty of Chemical and Life Sciences, Abdul Wali Khan University Mardan (AWKUM), Mardan, Pakistan
- Department of Genetics, Institute of Hydrobiology, University of Chinese Academy of Sciences (UCAS), Wuhan, PR China
- Department of Chemistry, Lahore College for Women University, Lahore, Pakistan
- epartment of Genetics, State Key Laboratory of Hybrid rice, College of Life Sciences, Wuhan University, Wuhan, PR China
Abstract
The significant rise in the number of diabetic patients worldwide, as well as the development of new insulin delivery techniques such as inhalation or oral administration which require higher dosages, are expected to increase the demand for recombinant insulin. Current manufacturing technologies will be unable to fulfill the rising demand for inexpensive insulin due to their production capacity limitations and high production costs. Production of therapeutic recombinant insulin requires a suitable host organism with adequate post-translational modification and refolding machinery. E. coli and S. cerevisiae have been used extensively to make recombinant human insulin for medicinal applications. However, transgenic plants are particularly appealing expression systems as they can be used to synthesize huge amounts of insulin for human medicinal purposes. Plant-based expression systems have the potential for high-capacity insulin synthesis at a minimal cost. The significant production of biologically active proinsulin in seeds or leaves with long-term stability provides a low-cost technique to develop proinsulin for both injectable and oral administration. Recently, stem cell therapy is being utilized for the treatment of diabetes, as these cells are capable of differentiating into insulin producing cells. With the advancement of regenerative medicine research for different chronic diseases, treatment for type 1 diabetes mellitus has been reported. The current review concentrates on several biotechnological attributes applied to the rapid and mass synthesis of biologically active insulin and its analogs in microbes, various types of stem cells and transgenic crops.
Introduction
An insulin shortage or the absence of proper cell responses to the available insulin can lead to diabetes mellitus1, 2. With diabetes and obesity reaching proportions of an epidemic in the developing world, insulin resistance and its associated symptoms are becoming more common3. Human insulin is a peptide hormone with a molecular mass of 5808 Da produced by the beta cells of the islets of Langerhans of the pancreas, and it is responsible for regulating the metabolism of glucose4. Some studies have demonstrated that a small amount of insulin may also be expressed in a subset of neurons in the central nervous system5. After a meal, the level of blood glucose rises and insulin is secreted in response6. When the insulin level falls, glucose is released by the liver into the blood7. Insulin was first reported in 1921 in extracts of the pancreas by the Canadian scientists Frederick G. Banting and Charles H. Best, while Nicolae C. Paulescu, a Romanian physiologist, was independently working on an insulin-containing substance he called “pancrein”8, 9. After the discovery of insulin by Banting and Best, work began to obtain purified insulin from the pancreatic extract. This was accomplished with the help of J. J. R. Macleod, a Scottish psychologist, and James B. Collip, a Canadian chemist10. Macleod and Banting shared the 1923 Nobel Prize in Medicine for their work on insulin11. Insulin was found to be a polypeptide in 1928 and its amino acid sequence was elucidated in 195212.
Insulin is composed of two protein chains, with 21 amino acids in the A chain and 30 amino acids in the B chain, totaling 51 in number, linked by disulfide bridges13. The pro-hormone proinsulin, from which insulin is derived, contains 74 amino acids, has a molecular weight of 5802 Da, and has an isoelectric point of 5.514. Proinsulin secreted by the beta cells is relatively inactive under biological conditions, but after cleavage in two places yields the two chains (B and A) of the active hormone insulin, and the biologically inactive C peptide15. The B (helical central segment) and A chains become linked together by disulfide bonds with an antiparallel linkage on the C-terminal helix15. The A and B chains are joined by a disulfide bridge which connects the C- and N-terminal helices to A and B with a central terminal (Figure 1). Proinsulin, insulin, and the C-peptide are stored in granules in the beta cells, which release insulin into the islet cells’ capillaries in response to a stimulus16. These capillaries empty into the portal vein, which carries blood from the intestines and stomach to the liver. After 30 minutes of exposure to hyperglycemia, the level of insulin in the blood will increase17. The healthy adult pancreas contains approximately 200 units of insulin, and the amount of daily insulin secretion into the circulatory system in healthy individuals ranges from 30 to 50 units 18.
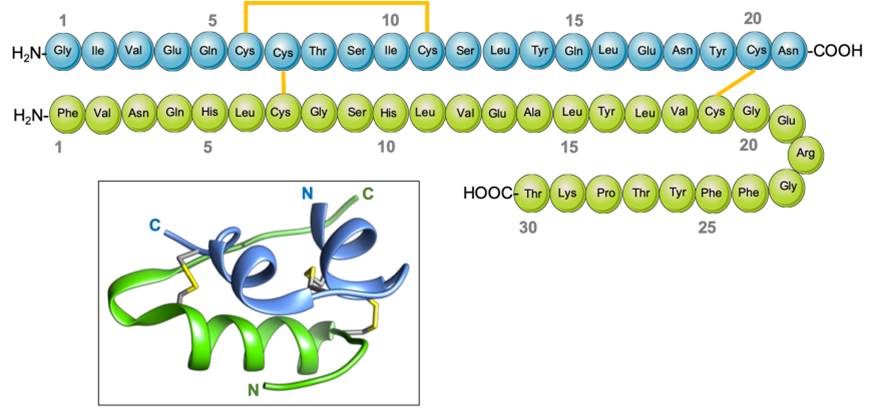
Comparative analysis of different expression systems used for production of human insulin. https://doi.org/10.6084/m9.figshare.16702819.v1
Various factors stimulate the secretion of insulin, but the most important is the glucose concentration in oxygenated arterial blood that perfuses the islet cells19. When the concentration of glucose in the blood increases, large amounts of glucose are taken up and metabolized by beta cells, and insulin secretion increases20. On the other hand, as the concentration of glucose in the blood decreases, insulin secretion decreases, though insulin is secreted in small amounts even during fasting21. Insulin secretion may also be stimulated by fatty acids, amino acids, several hormones, and keto acids (resulting from fatty acid metabolism) secreted by the gastrointestinal tract21. Insulin secretion is inhibited by somatostatin and by sympathetic activation of the nervous system, which includes the fight or flight response22.
Primarily, insulin acts to stimulate glucose uptake in liver, muscle, and adipose (fat) tissues, which play a significant role in nutrient storage and metabolism23. Like other protein hormones, insulin binds with specific receptors on the outer membrane of target cells, which causes metabolic processes to take place within those cells. A key action of insulin is to stimulate the translocation of glucose transporters to the cell membrane from within the cell24. Insulin is an anabolic hormone that promotes lipogenesis, glycogenesis, glucose uptake, and protein synthesis for skeletal muscle and fat tissues through the kinase tyrosine receptor pathway25. The prevalence of diabetes is increasing at an alarming rate, with estimates that the number of diabetic patients globally will reach 300 million by 202526. As a result, the demand for insulin will soar to about 16,000 kg/year, and the productivity of the existing insulin expression systems will be insufficient to satisfy these future market demands. More efficient insulin expression methods must be developed, in addition to novel insulin delivery routes, such as through ingestion or inhalation. The current article discusses the various approaches for the synthesis of insulin.
History of Insulin Production
Before insulin was discovered in 1921, patients with diabetes did not have long lives. The most successful therapy was to place diabetic patients on stringent carbohydrate-restricted diets. This could provide patients with a few more years of life, but could not entirely cure them. Patients have died of hunger as a result of strict diets consisting of only 450 calories per day27. Sir Edward Albert Sharpey-Shafer first proposed in 1910 that patients with diabetes had just one hormone lacking from their pancreas. Insulin is derived from the Latin word insula, which means "island"12. Frederick Banting, a young surgeon, discovered how to extract insulin from a dog's pancreas in 1921. The separated substance appeared to be "thick brown sludge," but they had no idea it would lead to life and hope for millions of diabetics28, 29.
Leonard Thompson, a 14-year-old child dying of diabetes in a Toronto hospital in January 1922, became the first person to receive an injection of insulin. His critically high blood glucose levels decreased to near-normal levels within 24 hours30. The Nobel Prize for Medicine was awarded to Banting and Macleod in 1923. Eli Lilly, a pharmaceutical company, began mass-producing insulin shortly after. Manufacturers produced several slower-acting insulins throughout the decades that followed, with Novo Nordisk Pharmaceuticals, Inc. introducing the first in 193631. Insulin from cows and pigs was used to treat diabetes for many years and saved millions of lives, though it was not optimal, as many people developed allergic responses to it32. The invention of DNA cloning by Stanley Cohen and Herbert Boyer heralded the beginning of genetic engineering, which allowed genes to be easily transferred across various biological species33. Their discovery led to the creation of various recombinant proteins with medicinal uses, including insulin and growth hormone. In 1978, bacteria were used to manufacture the first genetically engineered synthetic “human” insulin. Eli Lilly went on to market the first commercially accessible biosynthetic human insulin under the brand name Humulin in 1982, which was authorized by the FDA for medicinal use in humans34.
Production Steps of Insulin
The first insulin production method that will be discussed here involves the synthesis of proinsulin. An alternative two-chain method where the A and B chains of insulin are produced separately can also be used. Recombinant are used to produce adequate quantities of proinsulin. This recombinant protein is produced by incorporating proinsulin-producing plasmids into 35. The transformed cells are then grown on tryptic soy broth containing the antibiotic kanamycin monosulfate. The plasmid contains a kanamycin monosulfate resistance gene along with the proinsulin coding genes, so the transformed can survive in the broth. However, kanamycin monosulfate kills the cells that have not been transformed36.
After the transformed have been obtained, the next goal is to increase the cell count to initiate the production of proinsulin inclusion bodies. For this purpose, the original transformed cells that were grown in the tryptic broth and kanamycin monosulfate are then inoculated and grown in a bioreactor under controlled parameters to maximize insulin production. These parameters include temperature (37°C), pH (7), foam, and feed. The oxygen level is kept at a tension level of 30% and it is maintained by adjusting the quantity of glycerol-feeding37.
Fermentation and Parameters
In this method, six 200ml test tubes are used to grow 0.5g of the initial transformed cells in 1lL tryptic soy broth solution with 0.5g of kanamycin monosulfate. After the cells have been left to grow in the medium for 24 hours at 37°C they are inoculated in a bioreactor to promote growth and production of proinsulin38.
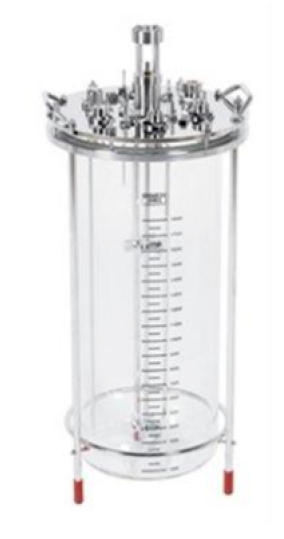
BioNet Reactor used for fermentation to produce human insulin. https://doi.org/10.6084/m9.figshare.16702825.v1
After 24 hours, the transformed has consumed and depleted all the nutrients in the test tubes, hence they are placed in the bioreactor to support further growth. This bioreactor has a total volume of 23L and a working volume of 16L. 1L of and depleted growth medium is taken and mixed with 9L of fresh growth medium in the bioreactor (Figure 2). Now, these cells will receive carbon from glycerol and yeast, nitrogen from ammonium sulfate and thiamine, and inorganic nutrients from potassium dihydrogen phosphate and dipotassium phosphate, which also act as buffers to maintain pH. Trace elements will be provided by sodium citrate, magnesium sulfate, and a vitamin solution39.
The conditions within the bioreactor are monitored by biosensors. The reaches its maximum growth within 28 hours, after which it is removed from the surrounding medium through centrifugation.
Cell Isolation by Centrifugation
Cell isolation is the first step in down streaming of the insulin made by transformed cells. This process is also referred to as cell harvesting because the proinsulin inclusion bodies are harvested using both filtration and centrifugation. Since has the highest density of all the components in the growth medium, the bacterial cells settle to the bottom after centrifugation at 7500 x g (8185 rpm) for 10 minutes. Then the supernatant is discarded and the dense mixture left behind is further processed since it contains a higher concentration of bacterial cells40.
Cell Lysis by Homogenization
The proinsulin inclusion bodies present inside the cell contain insulin precursor products in the form of proinsulin fusion proteins. Since they are present in dense aggregates, they are protected from being processed into the soluble form within the cytoplasm. To release these inclusion bodies, various methods of cell membrane disruption are available. In this particular process, high-pressure homogenization with a blade-type homogenizer, and chemical alkali treatment are used41.
The bacteria and medium are injected into the chamber of a high-pressure homogenizer with intense speed, and as a result, when the mixture encounters the blade present within the chamber, a condition of high turbulence and shear is created leading to compression, acceleration, and a pressure drop. The cell membrane is disrupted due to these forces, releasing the cytoplasmic contents. However, the proinsulin molecules remain intact. The pressure provided by the homogenizer is 45,000 PSI with a flow rate of 150 ml/min. Therefore, it can homogenize a 3L mixture of cells and medium in 20 minutes. After homogenization, the proinsulin must be separated from cell debris and intracellular material42.
Inclusion Body Separation by Centrifugation
After the have been lysed, the inclusion bodies need to be isolated from the cell debris. For this purpose, centrifugation can be used for reverse osmosis. Since the proinsulin inclusion bodies are dense, they will sink to the bottom. However, the speed of centrifugation must be higher (15000 x g for 30 minutes) than before, since the inclusion bodies have a lower density than the intact bacterial cells. After centrifugation, the supernatant is discarded while proinsulin and some impurities remain in the tube37.
Solubilization of Inclusion Bodies
After the separation of inclusion bodies, proinsulin is in an insoluble form and therefore must be solubilized. This is accomplished through the addition of denaturing agents such as urea or guanidium hydrochloric acid, which will release the fusion proteins. This process is followed by the addition of either β-mercaptoethanol or DTT, which are reducing agents, to break the disulfide bonds present within the proinsulin fusion proteins.
In the traditional proinsulin procedure, after solubilization, a cleavage step for the preparation of proinsulin is performed. This step can also be performed later in the processing. It involves adding cyanogen bromide and 70% formic acid to cleave the peptide linker between proinsulin and its fusion protein partner43.
Sulfitolysis
Sulfitolysis first involves breaking the disulfide bonds by adding reducing agents. These bonds get broken during solubilization or other earlier steps of purification. The process of sulfitolysis is performed along with 6 hours of solubilization, and 0.8M NaSO and 0.3M NaSO*HO are added to facilitate oxidation and maintain the unfolded form of proinsulin. Sulfite (SO) ions are added to cysteine molecules, which prevents the formation of incorrect disulfide bonds44. All of the cysteine residues in the proinsulin molecule have sulfite ions added. However, the ZZ tail has no cysteine residues, therefore it remains unaltered and is utilized in further downstream processes.
Additional Separation
Before renaturation, the impurities and reagents from solubilization and sulfitolysis must be removed via centrifugation at 17700 x g for 33 minutes45.
Dialysis
This process is used to remove the previously used denaturants and dissolved reagents without chemically modifying the fusion protein product. It involves the addition of buffers such as 10mM Tris-HCl (4 repetitions) to remove reagents including urea, DTT, and β-mercaptoethanol, and initiate the refolding process of the proinsulin fusion protein46.
Renaturation
The process of renaturation involves the correct folding of proteins, which depends heavily upon the correct formation of disulfide bonds. Renaturation is carried out for 20 hours at 4°C with the addition of 1M glycine-sodium hydroxide buffer (pH 10.5 or higher) and β-mercaptoethanol at an 18:1 molal ratio to the fusion protein. There are several methods available. Two commonly used methods are (1) the use of oxidative buffers such as low molarity Tris-HCl or glycine-sodium hydroxide to oxidize the reduced proinsulin, or (2) conversion of the proinsulin to the S-hexa-sulfonated form via sulfothiolysis using sodium sulfite, followed by the addition of redox reagents such as cysteamine, GSH or cysteine couples47.
Correct folding is the critical factor determining the yield of proinsulin products. Factors to optimize refolding include using an accelerated oxidation rate, basic pH of 9, and a high concentration of redox reagents. Even under optimized conditions, the yield is 60% to 70%. Therefore this step of the production process requires further research48.
Volume Reduction
After renaturation, the reagents and buffers that were used need to be removed so that the proinsulin product can be isolated. This can be done by the addition of weak acid for adjustment of pH followed by centrifugation at 17700 x g for 33 minutes. An alternative method can be sedimentation49.
Affinity Chromatography
It has been estimated that more than half of the cost of insulin production is spent on downstream purification processes rather than the growth of transformed bacteria. Therefore, purification techniques need to be time- and cost-effective, and provide a high yield of product that is pure enough for human use. This process involves volume reduction via site-specific cleavage chromatography of insulin from the proinsulin method50.
This method involves the purification of ZZ-R proinsulin through IgG affinity chromatography using an IgG-Sepharose column. It involves the use of acetic acid at pH 8 as the supernatant, which is centrifuged for 20 minutes before being used. 50ml of the solution is loaded at a flow rate of 3ml/min, which means that approximately 2.5g of ZZ-R proinsulin will be passed through the HR16/10 diameter column, which contains 12ml of IgG-Sepharose. The solution is added a total of 6 times, and the last three loads use 10mM of sodium acetate at pH 851.
The results of affinity chromatography are observed using SDS-PAGE on a homogenous 12% gel. It involves the use of size exclusion chromatography with a Superdex 75 PC3.2/10 column with the addition of 200 mM sodium phosphate buffer at a flow rate of 100ml/min. ZZ-R proinsulin is resistant to degradation by proteases when passed through affinity chromatography. It is then further purified by size exclusion chromatography, whose recovery rate is 70%. Most of the recovered proinsulin is a monomer, while other forms of ZZ-R proinsulin are also present in small quantities52.
Site-Specific Cleavage
After IgG chromatography, the purified ZZ-R proinsulin undergoes ultra-filtration which reduces its volume by 5 times, increasing its concentration to 12ml/min. Ultra-filtration is followed by cleavage of proinsulin into the C-peptide and insulin using trypsin and carboxypeptidase B. Trypsin is used for the breakdown of protein in the digestive system while carboxypeptidase is used for cleavage of proinsulin at a specific site to convert proinsulin into native insulin and the C-peptide. About 1:1000 by mass of trypsin to ZZ-R proinsulin is used, while the quantity of carboxypeptidase B is double that of ZZ-R proinsulin. The enzymatic reaction is allowed to proceed for 30 minutes, after which it is stopped by the addition of trifluoroacetic acid, carboxylic acid, and a common buffer at a pH of 3. 20% acetonitrile is also added. The resulting solution is stored at 4°C before further purification using reverse-phase chromatography43, 51.
Reverse-Phase High-Performance Liquid Chromatography
Reverse-phase high-performance liquid chromatography (RP-HPLC) is used to separate the C-peptide and human insulin. RP-HPLC is a common method used to analyze insulin products since it can separate insulin into its different species, and the use of high pressure increases the speed of the process and purity of the product. RP-HPLC involves a non-polar stationary phase and a polar mobile phase. Since insulin is non-polar and large, it adheres to the stationary phase column whereas the mobile phase contains methanol or acetonitrile in a buffer solution to analyze the insulin. The wavelengths used for the detection of insulin are 190 to 220 nanometers. Conveniently, both the chains of insulin can be separated using this method. For the A chain, trifluoroacetic acid is used while for the B chain, formic acid is used. However, to separate the C-peptide, the best technique is gel chromatography with 1 M acetic acid as a buffer37, 53.
Polishing with Zinc Complexation and Saline Dilution
The goal during insulin administration is to quicken its activation time and prolong the peak hours. When using regular insulin, R-insulin, the activation time is 30 to 60 minutes, while it reaches a peak after 2 to 4 hours of administration. This means that approximately 4 to 6 injections are needed daily. The primary methods to prolong the activity of insulin are by inhibiting its immediate use by cells, preventing the liver from removing it, and stabilizing it in the bloodstream54.
Eli Lilly and Co. have devised a method that requires only daily 2 insulin injections for people with an active lifestyle. They used zinc ions to form crystal complexes with insulin, named 2Zn insulin, which have a hexagonal configuration while exhibiting axial symmetry, hence slowing the release of insulin to the body and preventing its immediate use by cells55. Cobalt can also be used for this purpose. These metallic ions form weak ionic bonds with insulin, causing insulin molecules to congregate and form suspended crystals. The formation of crystals is achieved through batch crystallization, and the solution is stored at 4°C. The better and more regular the crystal, the longer it can remain active in the bloodstream56.
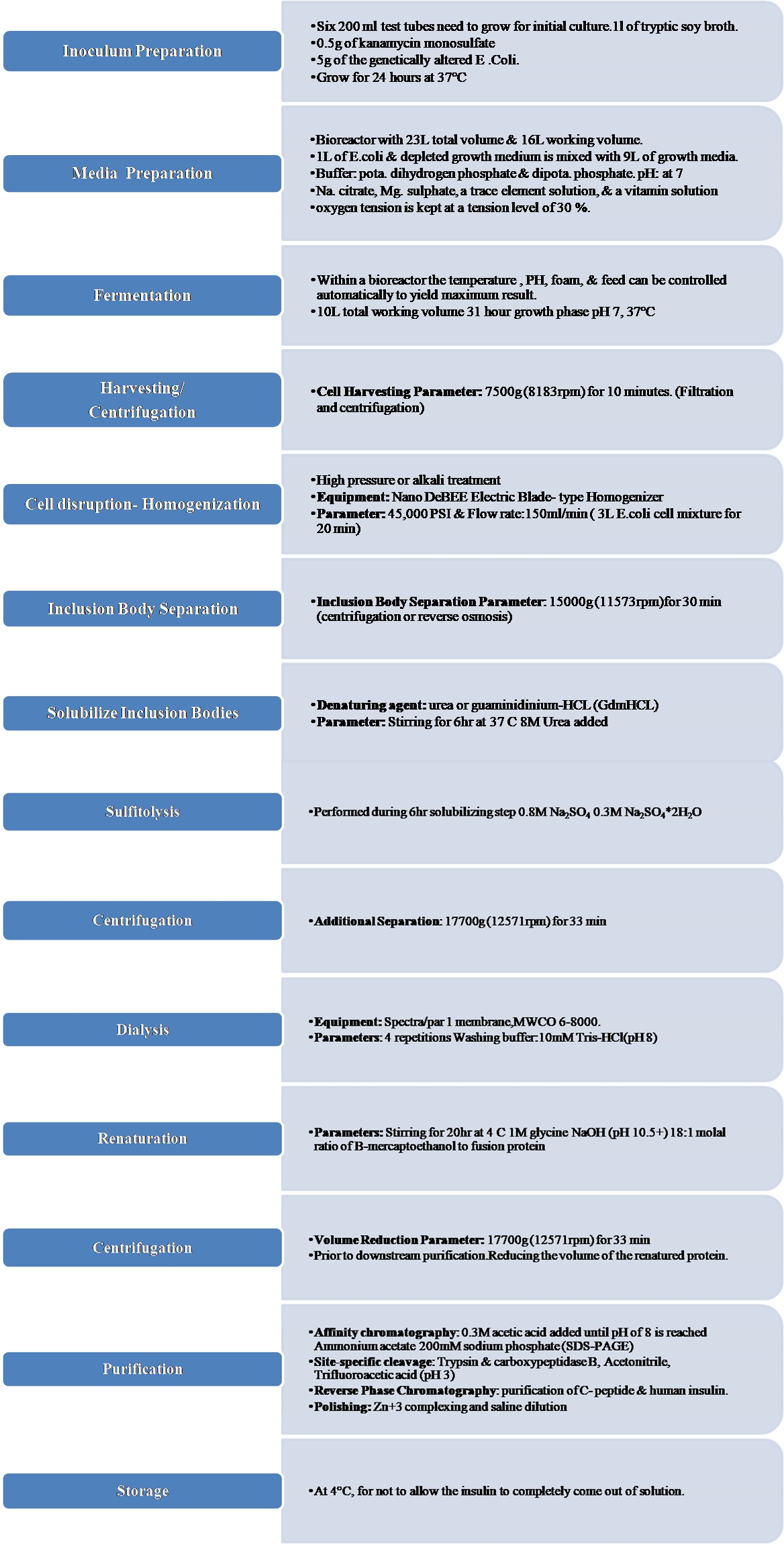
Diagrammatic representation of protocol involved in human insulin production. https://doi.org/10.6084/m9.figshare.16702828.v1
Moreover, crystallization with zinc can help alleviate zinc deficiency, which is commonly found in people with diabetes. Additionally, insulin is naturally stored as zinc hexamers in the beta cells of the islets of Langerhans. Commonly, 70% of the complexed crystalline insulin is mixed with 30% monomeric insulin, which allows for immediate use of the monomeric insulin by the body, while the complex insulin is released slowly over an extended period27, 57. The step-by-step procedure for human insulin production is illustrated in Figure 3.
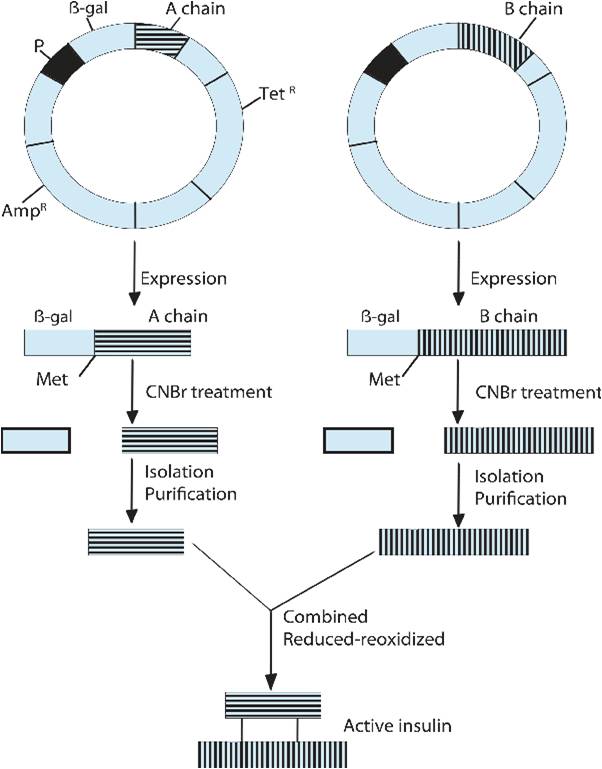
Mechanism of producing human insulin by utilizing chain method
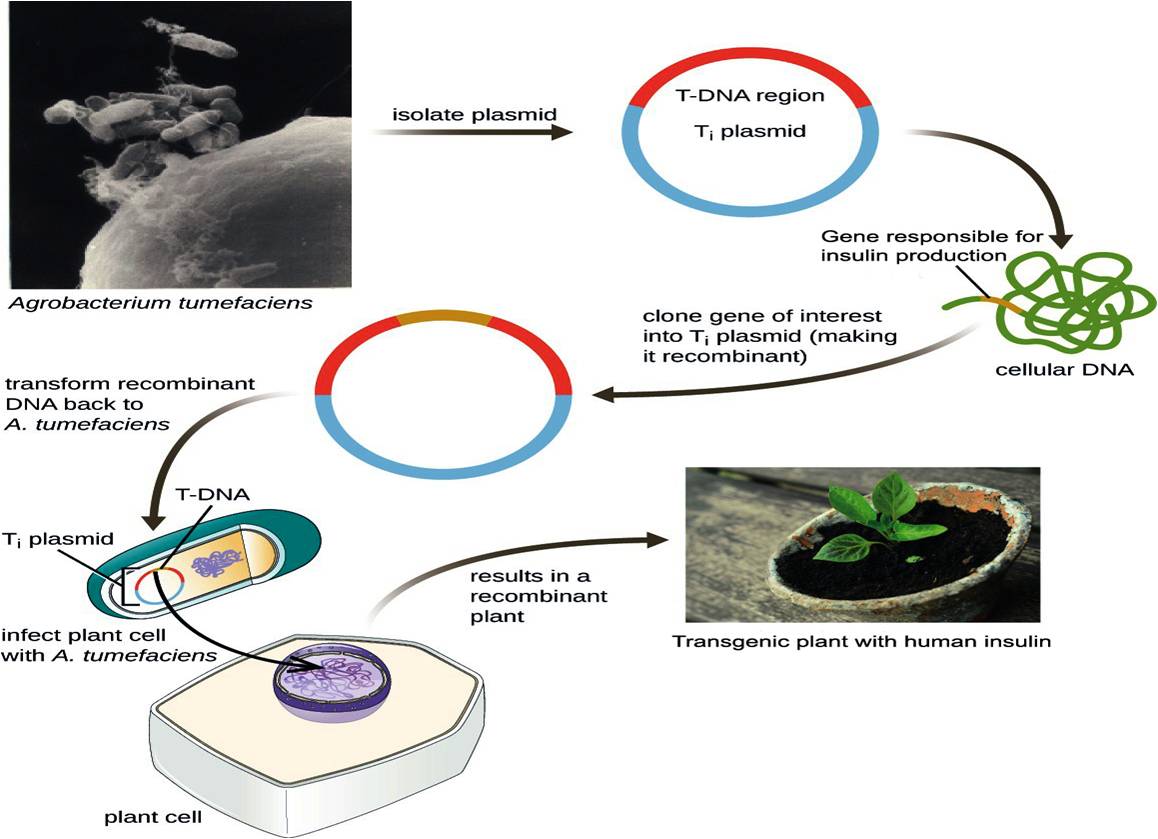
Mechanism involved in developing Agrobacterium mediated transgenic plant for human insulin production. https://doi.org/10.6084/m9.figshare.16702834.v1
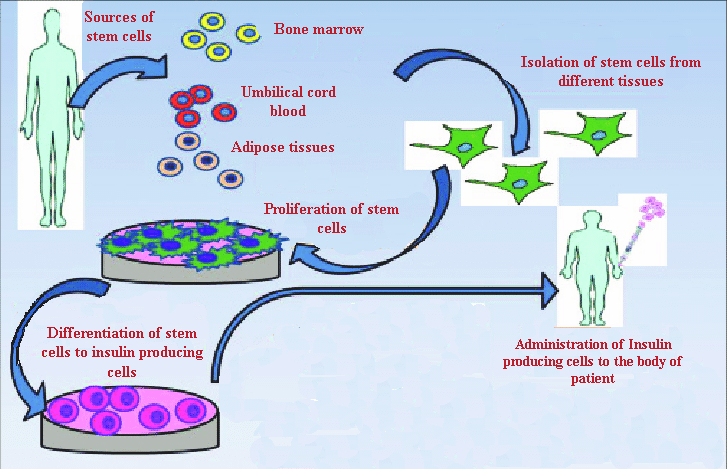
Representation of mechanism involve in isolation and differentiation of various types of stem cells to human insulin producing cells
Alternative Methods for Insulin Production
The second commercial method for insulin production is the two-chain method, in which the A chain and the B chain of insulin are produced separately and then fused (Figure 4). Here, these 2 polypeptides are cultured in bacteria in two different fermenters and then purified. The purified A and B chains are then incubated under oxidizing conditions to form the disulfide bonds that are present in human insulin37.
Bio-production Steps
Gene Isolation
Complementary DNA (cDNA) molecules encoding chain A and chain B are obtained from human insulin mRNA using reverse transcription34. The cDNAs of both chains are amplified by PCR.
Insertion into the Plasmid
Two plasmids are cut using restriction enzymes to insert the DNA sequence for the A chain or the B chain separately. Each chain is extended with an ATG initiation codon on the 5’ terminus to begin the translation process, while the termination signal is present on the plasmid at 3’end of the restriction sites60. The restriction sites of EcoR1 and BamH1 contain one of the chain genes in both plasmids. The plasmid also contains a lacZ gene, which encodes for β-galactosidase, allowing for colony screening. Specific DNA ligases are added to bind the inserted chain gene into the plasmid61.
Transfection
The entry of recombinant plasmids into bacterial cells is called transfection. Different techniques can be used for the transformation of such as treatment with CaCl or electroporation techniques. After the plasmid’s entry, the cells become transformed62.
Medium Preparation
LB broth is used as a culture medium for . It is first dissolved and the solution is autoclaved for sterilization, and then ampicillin and lactose are added. The medium is inoculated with transformed cells57. STR bioreactors are used for fermentation of the two strains encoding the insulin chains. The bioreactors are sterilized and the pH, pOprobe, condensers, and air inlet are calibrated63.
Bioreactor Fermentation
Shake flasks are used for small-scale fermentation of recombinant cells encoding the A and B chains in the enriched medium, which are then used for the large-scale fermentation process63. The ampicillin resistance and lacZ genes present on the plasmids are used for the identification of successfully transformed cells, as they show resistance against the ampicillin present in the growth medium and encode β-galactosidase. These successfully transformed cells are retained for replication under optimal conditions and then transferred into a bioreactor for commercial production. The A and B chains are synthesized as their respective strains replicate in separate fermenters64.
Crude Product Isolation
The bacterial cells are removed from the bioreactor tank and must be lysed with one of several methods, such as enzyme digestion, sonication, or freezing and thawing of cells. The use of lysosome enzymes is preferred for large-scale operations, as it digests the bacterial outer layer to release the insulin into the surrounding media, so detergents can later be added to remove the cell wall64.
Purification
Cell components are separated from the two desired insulin chains. Gel filtration and ion-exchange chromatography methods are used to remove impurities64.
Insulin Chain Isolation
The isolated purified protein has an insulin chain fused with β-galactosidase, as it has been linked with the gene incorporated into the plasmid and translated along with it. Cyanogen bromide is used to separate the insulin chains from β-galactosidase, as it splits the protein at the methionine residue which begins the β-galactosidase protein65.
Chain Joining
The insulin chains (A and B) are treated with sodium dithionite and sodium sulfite to form the disulfide bonds that bridge the chains. This whole process is called reduction-reoxidation, and it is induced by β-mercaptoethanol and air oxidation to synthesize human insulin58.
Reverse-Phase High-Performance Liquid Chromatography
RP-HPLC is performed to remove the remaining impurities or reagents. The purified, active human insulin can then be packed and sold by the industry66.
Different Expression Systems
Recombinant human insulin is usually commercially produced in or . With the expression system, the inclusion bodies of insulin precursors (IPs) are produced, which are then solubilized and refolded to create active insulin. The yeast expression system yields the IPs in culture-supernatant, therefore it is often selected for commercial production. Several other insulin expression systems are being studied37. Some yeast strains are being manipulated to explore insulin production. Additionally, many mammalian cells and plants are under investigation for their suitability for large-scale production67. To fulfill the world’s requirement for insulin of 16,000 kg per year, novel and efficient expression systems must be developed37, 68.
The expression system was the first expression system used to produce human insulin in 1978, with the use of recombination technology in the two-chain method. This expression system is preferred for the large-scale production of R-insulin, as grows rapidly on cheap media, is easy to handle, and can be genetically manipulated69. However, several aspects limit its usefulness, including loss of plasmids, development of antibiotic properties, lack of post-translational modifications (PTMs), inclusion bodies’ accumulation within the cells, improper folding, proteolytic digestion, and poor secretion that makes protein recovery difficult70. Further research and the development of new technologies have tried to solve these problems. Some of the approaches are mentioned here.
Recently a new PCR-based approach was used for the cloning of human insulin, eliminating several steps such as affinity tags, tedious protein renaturation, inclusion body recovery steps, and the enzymatic cleavage of the C-peptide of insulin, making this method novel and more efficient45.
In 2019, Zieliński. reported the construction of the novel pIBAINS expression vector and the establishment of 20 novel strains, which provide greater efficiency of recombinant insulin production per liter of medium46.
Yeast Expression Systems
Yeast is another commercially-used expression system for heterogeneous protein production with recombinant technology because of its PTMs. Such PTMs include acylation, phosphorylation, and N- and O-linked glycosylation of proteins74. Yeast can easily be scaled up in bioreactors and its use is cost-effective, which makes it a potential candidate as an expression system for the production of recombinant insulin. However, a factor of concern when using yeast as an expression system is that proteins synthesized in yeast have high-mannose N-glycosylation. This shortens the half-life of recombinant proteins and activates an immune response in humans because proteins with this modification are considered foreign antigens. Thus, yeast has to be humanized before use by modifying its N-glycosylation pathways to create less immunogenic products for humans75.
has been used commercially as a yeast expression system for insulin since the 1980s by creating proinsulin, which links the A and B chains via a short synthetic C-peptide37, 64. The α-factor signal sequence helps in the synthesis of the chains and increases proinsulin expression to 80 mg/ml76. Proinsulin is converted to active insulin by a trypsin-mediated transpeptidation reaction with a threonine ester. Other insulin analogs synthesized using yeast include:
The high cell-density, methylotrophic yeast is also used commercially for insulin production80. A special feature of this yeast expression system is the presence of the methanol-inducible alcohol oxidase-1 promoter (AOX-1), which increases the cell density under simple cultivation strategies to help in the large-scale production of recombinant proteins. Unlike does not hyperglycosylate its secreted heterogeneous proteins, proving advantageous for use in the creation of human therapeutics81. Both yeast expression systems do carry out high-mannose N-glycosylation, but in , the oligosaccharide chain consists of 8-14 mannose residues/chain, which is far shorter than the 50-150 mannose residues/chain added by . Heterogeneous protein expression attained by is approximately 30% of its total cell protein, which is much higher than S. cerevisiae82. Such characteristics make an attractive expression system for use in the commercial production of recombinant human insulin and its analogs. The highest yield of insulin precursor, 1.5 g of IP/L of culture broth, was recorded from the expression system83.
Transgenic Plants
Of recent interest in the field is the production of recombinant or heterogeneous proteins from transgenic plants, which have the advantage of low cost and high protein quality84. Plants do not have human pathogens and because they are eukaryotic, their PTM machinery is more similar to humans than that previously described in yeast85. The mechanism involved in producing human insulin in transgenic plants is illustrated in Figure 5.
With recent experimentation, the human insulin gene has been successfully expressed in oilseeds of the plant37. This plant has a short generation time of almost 6 weeks and can easily grow under laboratory conditions with limited sunlight. The genome of is completely known, making it easier to perform further research on recombinant insulin production. Each plant is capable of generating approximately 10,000 to 30,000 seeds86. The insulin is expressed in subcellular organelles of plant-like oil bodies which, along with a high amount of protein expression, makes its recovery easier. Inside the oilseeds, the oil bodies are encapsulated by a hydrophobic tri-acylglycerol phospholipid membrane, and their outer wall is made up of oleosins87. These oil bodies can be easily separated from other seed components by liquid-liquid phase separation methods, reducing the need for chromatographic steps to purify the insulin. Experimental results showed that the insulin accumulation in transgenic seeds is up to 0.31% of the total seed proteins88. Trypsin digestion is used to cleave the oleosin from the oil bodies, which also purifies and matures the active insulin via simple purification methods. The use of seeds as an expression system is also advantageous because the insulin can be stockpiled until it is required.
Tobacco and Lettuce Plants
The tobacco plant exhibits higher seed germination and survival rates, while the lettuce plant is widely consumed across the globe and is a very attractive expression system89, 90. The chloroplasts of these plants are used to synthesize proinsulin. The insulin chains (A and B) and the C-peptide become fused with subunits of cholera toxin-B91, 92. The reported data show that old leaves of tobacco plants contain almost 47% of proinsulin out of their total leaf proteins91. In old leaves of lettuce, up to 53% of total leaf proteins were recorded as proinsulin91. The extracted insulin is found to be very stable and has up to 98% purity when cleaved by Furin protease treatment to release the insulin peptides. Oral delivery of plant cells also shows similar results as commercially available insulin. The recorded yield of proinsulin by these plants is 3 mg/g of leaves, which indicates that one acre of such plants could produce 20 million daily doses of insulin every year37, 72.
Strawberry Plant
Strawberry ( Duch.) is one of the world's most significant and popular fruits and contains several vitamins, minerals, anthocyanins, and essential amino acids that contribute to human health93, 94. However, the edible nature of the strawberry makes it a useful plant for insulin production, as it can be used as a vehicle for oral administration. Also, strawberries contain levulose, which is quickly absorbed and decomposed in the body and does not affect people with diabetes95. Therefore, the strawberry should be studied to determine if oral insulin administration is a viable option for people with diabetes. According to Mendel's rules of inheritance, if a gene is delivered into the nucleus of a plant cell, at least one-fourth of the plant will be non-transgenic in the following generation96. However, the presence of runners in strawberry plants, which are clones of the mother plant, allows each generation to have a mother plant95. Because of these qualities, the strawberry plant is a suitable expression system for human proinsulin gene transformation and recombinant protein production.
In a recent study, Taviziinfected strawberry explants (leaves, petiole, and buds) with and that contained insulin-producing genes. The hairy roots that grew as a result of the treatment with were subcultured in a bioreactor for several days, while the explants infected with were micropropagated. The transmission and expression of the insulin genes in the hairy roots and regenerated plants were analyzed through PCR, RT-PCR, and ELISA. Additionally, the proinsulin was purified from the transgenic plants and hairy roots and injected into diabetic rats. 60 ng of the purified insulin was able to significantly reduce the blood sugar levels in these rats95.
Role of Stem Cells in the Treatment of Diabetes
Stem cells are specialized cells that eventually develop into the tissues and organs of the body. Throughout its life, the body relies on stem cells to repair damaged tissues and cells that are lost regularly, as they can self-renew and differentiate. Embryonic stem cells (ESCs), mesenchymal stem cells (MSCs), and induced pluripotent stem cells (iPSCs) have all been developed into insulin-producing cells (Figure 6)2, 97.
Embryonic Stem Cells (ESCs)
ESCs can develop into endoderm, mesoderm, and ectoderm cells and are extracted from blastocysts. When ESCs are transplanted into diabetic mice, they can develop into insulin-producing cells, which can release insulin in response to glucose stimulation and restore proper blood glucose levels98, 99. Human ESCs can also be differentiated into endocrine cells, however, this carries the risk of promoting tumor formation100.
Induced Pluripotent Stem Cells (iPSCs)
Like ESCs, iPSCs have an indefinite capacity for self-renewal and the ability to differentiate into a wide range of cell types. Undifferentiated iPSCs can be maintained as cell lines, which holds tremendous potential for disease modeling and the development of autologous cell therapies101. iPSCs generated from mouse skin fibroblasts were able to develop into β-like cells, comparable to the natural, endogenous insulin-secreting cells, and helped diabetic mice regulate hyperglycemia102. Human ESCs and iPSCs have been developed into mature pancreatic cells capable of secreting insulin and C-peptide103. Insulin-producing cells were also grown from iPSCs using small chemicals and growth factors104.
Mesenchymal Stem Cells (MSCs)
MSCs can be extracted from adipose tissue, mobilized peripheral blood, fetal liver, periodontal ligament, umbilical cord blood, placenta, fetal lung, dental pulp, umbilical cord, synovial membrane, endometrium, compact bone, trabecular and dental pulp, synovial membrane, endometrium, periodontal ligament, and trabecular and compact bone105. MSCs can differentiate into mesodermal, endodermal, and even ectodermal cells when cultured under the right circumstances106. They can secrete growth factors and immunoprotective cytokines during transplantation107. MSCs have also been injected directly into the pancreas as niche-providing cells, which helped relieve diabetic symptoms in animal models by improving metabolic regulation, counteracting autoimmunity, boosting islet engraftment and survival, and acting as a source of growth factors and cytokines108. MSC injections enhance pancreatic function while also alleviating symptoms such as diabetic foot, nephropathy, and neuropathy. Although MSCs appear to have a significant impact, it is more likely to be a niche effect than genuine regeneration109.
Bone Marrow-Derived Mesenchymal Stem Cells
Human bone marrow (BM)-derived MSCs can be maintained for up to 44 weeks without losing their morphological, phenotypical, functional, or karyotype features110. MSCs from the BM suppress the immunological response of T cells towards freshly generated β cells111. As a result, stem cell therapy may be the most effective treatment for patients with type 1 diabetes. Stem cells migrate to the injured area, develop, and initiate structural and functional repair, which aids in the treatment of diabetes and the normalization of insulin levels. Mouse bone marrow cells developed into functional β cells in an experiment112. In another investigation, iPSCs from BM-MSC rats were shown to cure chronic hyperglycemia in diabetic rats.
Adipose-Derived Stromal Cells (ADSCs)
Adipose-derived stromal cells (ADSCs), also known as adipose tissues isolated from human lipoaspirates, can be obtained in high quantities and have differentiation capabilities comparable to BM-MSCs. When AD-MSCs were grown in a fibroblast growth factor-containing medium, they produced markers such as Isl1 mRNA, which are required for the formation of pancreatic islet cells113. Several studies have shown that AD-MSCs from the mouse epididymis can develop into cells that express PDX1, Ngn3, NeuroD, Pax4, Glut2, and produce insulin and C-peptide114. After 38 days of co-culture with islet cells, AD-MSCs were shown to be differentiated into iPSCs115. Compared to islet transplants alone or co-transplantation of islets and differentiated BM-MSCs, combining differentiated AD-MSCs and islet cells resulted in better diabetes recovery116.
Human Umbilical Cord Blood-Derived Mesenchymal Stem Cells
MSCs from umbilical cord blood and unrestricted somatic stem cells from hUCB can develop into iPSCs with the same cell markers and characteristics as MAPCs (multipotent adult progenitor cells)117. After incubation in a medium supplied with no particular cytokines or growth factors besides fetal calf serum, UCB cells express genes necessary for differentiation into pancreatic endocrine tissue, including Isl1, PDX1, Pax4, and Ngn3113. and , insulin and the C-peptide were released by iPSCs generated from UCB-MSCs in response to a glucose challenge. After intravenous injection of hUCB-MSCs into 25 NOD type 1 diabetic mice with insulitis, glycemic profiles improved along with histological improvements in the islets118. UCB-MSCs are widely accessible, have minimal risk of immunological rejection, and show improved capabilities for growth and differentiation into iPSCs, making them a viable choice for the treatment of diabetes.
Comparative Analysis
Humanized gene expression is better in eukaryotic systems than in prokaryotic systems. The human insulin gene, which is eukaryotic, cannot be immediately transferred to a prokaryotic expression system, because the gene contains introns that must be spliced before translation, followed by PTMs to create the active insulin product. Instead, cDNA of the desired gene is created and then injected into a prokaryotic cell. has historically been the favored prokaryotic expression system for large-scale recombinant protein production because of its ease of genetic modification, cost-effectiveness, and high growth and recombinant protein synthesis rates119, 120. However, this expression system comprises a large number of uncommon codons and has a high incidence of translational mistakes such as amino acid substitutions, premature translation termination, and frame-shift mutations. In the case of therapeutic proteins like insulin, such mistakes might degrade the quality, causing an immunogenic reaction in humans121. PTMs, loss of plasmids, antibiotic properties, and the downstream procedures to extract the required proteins from chaperones are some of the drawbacks of employing an expression method.
Humanized yeast expression systems, which use single-celled organisms with eukaryotic post-translational modification processes, can be employed to overcome the aforementioned constraints. On a large scale, yeast can be readily maintained using a basic medium. Among yeast species, plays a crucial role, particularly in the production of proinsulin analogs. With naturally occurring plasmids, it has been described to have strong related promoters. It only produces a few proteins. Regardless of its high copy number and the presence of LUE2, yeast episomal plasmids are most commonly utilized for manufacturing122. is used to produce Insulin Aspart, a fast-acting insulin derivative. , on the other hand, is a better choice for industrial-scale production due to its plasmid degradation and lesser extent of hyperglycosylation. In both bacterial and yeast expression methods, the total space-time yield is similar, although yeast, particularly , is more appropriate123.
As transgenic plants do not contain human diseases, they are thought to be a safer expression method for human insulin. seeds, tobacco and lettuce plant leaves, and strawberry root hairs are among the transgenic plants being studied for use in proinsulin production. Physiologically active insulin can be readily isolated from subcellular oil bodies of seeds using liquid-phase chromatography124. At a larger scale, enzymatic purification can be employed. The shockability and low cost of seeds as a bioreactor are the two most essential advantages. However, difficulties with protein stability remain a key concern when utilizing plant expression systems. To prevent problems like these, tobacco and lettuce plant leaves were chosen as proinsulin bioreactors125. In their global usage, tobacco provides protection against food-chain contamination, while lettuce does not126. However, the chloroplast-based expression mechanism utilized in both plants has chloroplast glucan interference, nonhuman glycosylation, and may require PTMs. As a result, before employing such expression methods, changes to the production method are necessary127. Strawberry is another intriguing plant for proinsulin synthesis, and its root hairs are being studied as a bioreactor. It is, so far, the safest option, as there is no gene escape128. Unfortunately, it can induce allergic responses in people when consumed orally.
ESCs are a popular target for researchers because they are produced from unused or unfertilized embryos at fertilization clinics, but there are few limitations in utilizing these cells. They should only be utilized for clinical studies with the prior consent of the donor. However, in the majority of cases, cells from the embryo are acquired by destroying the embryo, which raises ethical concerns regarding the origin of life and the right to annihilate the embryo129. Human ESCs can be differentiated into endocrine cells, but they may also promote tumor growth130.
The lack of ethical issues and minimal probability of teratoma development are two advantages of utilizing iPSCs131. The most common method for transforming somatic cells into iPSCs is to use viral transfection of transcription factors. The use of harmful genomes, which can cause mutations and affect normal iPSC function, differentiation ability, and tumorigenesis, is an important drawback of this technology131. Similarly, MSCs seldom differentiate spontaneously in the host tissue. Thus, their therapeutic utility relies on the capacity to regulate their differentiation into functional cells with high efficiency and purity132. Additionally, MSCs can develop into undesirable mesenchymal lineages, which might restrict their therapeutic effectiveness133. A comparative analysis of the different expression systems that have been discussed for insulin production is provided in
Comparative analysis of different expression systems used for production of human insulin
Expression Systems |
Advantage |
Year of FDA Approval |
Limitations |
Advancements |
References |
---|---|---|---|---|---|
Prokaryotic Expression System | |||||
|
Versatility and cost-effectiveness for large scale production |
US FDA in 1982 |
Plasmid loss, high probability of translation errors which can cause adverse immunological responses in human |
Colony PCR strategies for similarity verification, strain modifications, codon optimizations and addition of chaperones |
|
Yeast Expression System | |||||
|
Eukaryotic model systemwhich enables the quality production and proper folding. |
US FDA in 2001 |
Plasmid degradation and Hyper-glycosylation on large scale. Time consuming purification methods. |
Modification in recombinant sequence of B chain cDNA to prolong the insulin effect duration. |
|
|
High cell density of Methylotrophic yeast with methanol-inducible alcohol oxidase-1 (AOX-1)promoter, do not hyper glycosylate insulin |
US FDA in 2009 |
High mannose structures and glycosylation heterogeneity can cause batch-to-batch variation |
Engineered strains for higher secretion levels, AOX1-based methanol-free protein manufacture, modified N-glycosylation machinery |
|
Transgenic Plants | |||||
|
Large amount of seed generation in 6 weeks, easy purification of oil bodies, no human pathogen, shockable |
Approval required |
Protein stability issues Inappropriate containment strategies |
Finding tissue-specific promoters to avoid toxicity |
|
Tobacco |
High number of leaves yield contains proinsulin, high purity protein with minimal PTMs requirement, less transgene containment. |
Chloroplast glycoproteins interference |
Transformed chloroplast, CTB-insulin fusion |
| |
Lettuce |
Long term stability in leaves, more leaf protein yield then tobacco plant |
Lack of regulatory approval due to food-chain contamination, Improper glycosylation |
Transformed chloroplast |
| |
Strawberry |
Oral consumption, using hairy roots as bioreactor |
lack of regulatory approval, unwanted allergic reactions |
No gene escape |
| |
Stem cells | |||||
Embryonic stem cells (ESC) |
Can differentiate into any cell type |
Approval required |
Ethical issues, Chances of immune rejection, genetic instability, teratocarcinomas formation |
Generation of pancreatic progenitor cells through ESCs |
|
Induced pluripotent stem cells (iPSCs) |
No ethical issues, less immune rejection chances, variable sources to derive iPSCs, can differentiate into variety of cell types |
Require reteroviruses to generate iPSCs, chances of cancer formation |
Use of non-viral reprogramming factors for generation of iPSCs |
| |
Bone Marrow derived Mesenchymal stem cells (BM-MSCs) |
Easy isolation, no ethical issues, can differentiate in variety of cell types |
Minimal differentiation potential, differentiate into unwanted mesenchymal lineages |
Use of nanotechnology and gene regulation enhanced the differentiation and proliferation ability of MSCs |
| |
Adipose-derived Mesenchymal Stem cells (AD-MSCs) |
No ethical issues, different isolation sources, high histocompatibility and low immune rejection |
Low multipotency and proliferative ability, genetic instability, apoptosis occurs in newly produced cells |
| ||
Human Placenta derived Mesenchymal stem cells (HP-MSCs) |
High proliferative and differentiation rate, Ease in extraction |
Use of nanotechnology to promote drug delivery and detection of transplanted cells |
| ||
Human Umbilical Cord derived Mesenchymal stem cells (HUC-MSCs) |
High proliferative and differentiation rate, 10 times more stem cells than those collected from bone marrow, Anti-inflammatory properties, anti-fibrotic potential, low risk of immune rejection |
Limited cells can be isolated for once from umbilical cord, less banks for storage of umbilical cords, require more time to graft |
|
Conclusions and Future Directions
The two conventional methods of producing insulin by recombinant technology are the proinsulin method and the two-chain method. The proinsulin method was developed more recently and is more reliable because it involves fewer steps than the two-chain method, making it effective and cost-efficient. Bacterial and yeast expression systems are generally preferred for commercial production, but novel organisms and transgenic plants have recently been introduced. In and the C-peptide of proinsulin is absent, which can be dealt with by certain genetic modifications in existing expression systems or by using new transgenic organisms like , , tobacco, strawberry, or lettuce. Additionally, transgenic plants can provide a higher level of protein expression. Even though human MSCs could be differentiated into functional pancreatic cells , the rate of trans-differentiation is limited, and the length of functional maintenance is challenging to assess. Considering the lack of defined protocols for the growth and production of insulin-secreting cells, clinical results are inconsistent. The use of novel approaches such as nanotechnology, signaling hormones, non-viral programming, or gene regulation of different types of stem cells can be utilized to develop insulin-producing cells that can serve as a useful treatment option for patients with diabetes. To achieve long-term stability, low-cost technologies should be promoted. Additionally, oral delivery of insulin is a safer method.
Abbreviations
:
:
:
AD-MSCs: Adipose-derived Mesenchymal Stem cells
AOX-1: Alcohol oxidase-1 promoter
BM-MSCs: Bone Marrow derived Mesenchymal stem cells
CNBr: Cyanogen bromide
CNS: Central nervous system
DTT: Dithiothreitol
:
ESCs: Embryonic stem cells
GI: Gastrointestinal tract
GSH: Glutathione
HP-MSCs: Human Placenta derived Mesenchymal stem cells
HUC-MSCs: Human Umbilical Cord derived Mesenchymal stem cells
IP: Insulin precursors
iPSCs: Induced pluripotent stem cells
LB: Luria Broth
:
PSI: Pounds per square inch
PTMs: Post-translational modifications
RE: Restriction enzyme
Rpm: Revolutions per minute
:
STR: Stirred tank reactor
T1DM patients: Type 1 Diabetes mellitus patients
Acknowledgments
The authors are thankful to the team of Big Bio for their support.
Author’s contributions
All the authors contributed equally in manuscript writing. J. Alyas, A. Rafiq, H. Amir and T. Sajid conceptualized study and wrote introduction of the manuscript. S. U. Khan, T. Sultana, A. Hameed and I. Ahmad, wrote about the insulin production and revised it critically. A. Ali and A. Kazmi designed and modified the figures, wrote manuscript and approved the final version of the manuscript for submission.
Funding
None.
Availability of data and materials
Not applicable.
Ethics approval and consent to participate
Not applicable.
Consent for publication
Not applicable.
Competing interests
The authors declare that they have no competing interests.